the Creative Commons Attribution 4.0 License.
the Creative Commons Attribution 4.0 License.
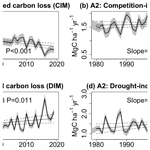
The impacts of elevated CO2 on forest growth, mortality, and recovery in the Amazon rainforest
Yitong Yao
Philippe Ciais
Emilie Joetzjer
Yujie Wang
Christian Frankenberg
Nicolas Viovy
The Amazon rainforest plays a crucial role in global carbon storage, but a minor destabilization of these forests could result in considerable carbon loss. Among the external factors affecting vegetation, elevated CO2 (eCO2) levels have long been anticipated to have positive impacts on vegetation, including the direct enhancement of both photosynthesis and productivity and increasing water use efficiency. However, the overall impact of eCO2 on the net carbon balance, especially concerning tree-mortality-induced carbon loss and recovery following extreme drought events, has remained elusive. Here, we use a process-based model that couples physiological CO2 effects with demography and both drought mortality and resistance processes. The model was previously calibrated to reproduce observed drought responses of Amazon forest sites. The model results, based on factorial simulations with and without eCO2, reveal that eCO2 enhances forest growth and promotes competition between trees, leading to more natural self-thinning of forest stands. This occurs following a growth–mortality trade-off response, although the growth outweighs the tree loss. Additionally, eCO2 provides water-saving benefits, reducing the risk of tree mortality during drought episodes. However, extra carbon losses could still occur due to an eCO2-induced increase in background biomass density, leading to “more carbon available to lose” when severe droughts happen. Furthermore, we found that eCO2 accelerates drought recovery and enhances drought resistance and resilience. By delving into the less-explored aspect of tree mortality response to eCO2, the model improvements advance our understanding of how carbon balance responds to eCO2, particularly regarding mechanisms of continuous competition-induced carbon loss vs. pulses of drought-induced carbon loss. These findings provide valuable insights into the intricate ways in which rising CO2 influences forest carbon dynamics and vulnerability, offering a critical understanding of the Amazon rainforest's evolution amidst more frequent and intense extreme climate events.
- Article
(2169 KB) - Full-text XML
-
Supplement
(1040 KB) - BibTeX
- EndNote
The intact Amazon rainforest influences present and future global carbon dynamics, accounting for a carbon sink of 0.42–0.65 PgC yr−1 between 1990–2007 (Pan et al., 2011) and containing 40 % of the world's tropical-forest aboveground biomass (Liu et al., 2015), a large carbon stock that is projected to be vulnerable to climate change (Boulton et al., 2022). Preserving this carbon stock is essential for regulating global CO2 levels and stabilizing the Earth's climate. As climate change progresses and CO2 levels rise, tropical rainforests may both increase carbon sequestration and become destabilized by climate risks. The impact of elevated CO2 (eCO2) on carbon sequestration can be separated into two components: direct effects related to higher leaf carboxylation rates, which may translate into a higher leaf area index, higher tree productivity, and increased biomass (known as CO2 fertilization), and indirect effects, i.e., partial stomatal closure and subsequently increased water use efficiency (WUE; CO2 physiological forcing) (Smith et al., 2020). In turn, higher leaf area increases transpiration and can increase water stress. However, the potential translation of increased individual-tree growth rates into biomass accumulation at the ecosystem level remains uncertain, given that eCO2 not only enhances carbon inputs at the ecosystem level but also amplifies carbon loss through growth–mortality trade-offs with higher growth, possibly leading to more competition between trees and higher mortality rates. Such “high gain/high loss” patterns reflecting a coupling between growth and mortality have been identified across spatial gradients (Needham et al., 2020; Stephenson and van Mantgem, 2005; Walker et al., 2021) and also seem to emerge in terms of temporal trajectories, with a parallel increase in growth and mortality, observed, for example, in the Amazon (Lewis et al., 2004) and other rainforests from long-term inventories (Hubau et al., 2020). For example, censuses of forest inventory plots within intact tropical forests in Amazonia have repeatedly revealed a faster increase in carbon losses from tree mortality that surpasses the increase in carbon gains attributed to both tree growth and new-tree recruitment, resulting in a decline in the net forest carbon sink (Hubau et al., 2020). Although a positive effect of eCO2 on increased tree loss has been hypothesized by McDowell et al. (2022), establishing a significant correlation between carbon loss and eCO2 has proven to be elusive (Hubau et al., 2020).
Compared to the research concentrating on vegetation productivity in response to eCO2, less attention has been directed toward the response of carbon loss, although minor disruptions of mortality rates in high-biomass systems, such as the intact Amazon rainforests, could trigger substantial carbon loss. An increase in tree mortality can reduce plant carbon residence time and consequently counteract the enhanced productivity (Friend et al., 2014). A comprehensive understanding of the response of tree mortality to eCO2 is thus crucial for unraveling forest biomass carbon dynamics. Carbon loss can arise from internal ecosystem processes, such as competition-induced self-thinning, the death of demoted trees, and the death of individual large trees (forming gaps), and external drivers, such as extreme climate events, insects, and pathogens (Fig. 1). McDowell et al. (2018) outlined two potential mechanisms underlying the connection between eCO2 and an increased tree mortality rate. First, enhanced individual-tree growth rates could accelerate self-thinning due to increased competition. Second, eCO2 makes trees larger and more vulnerable to the external environmental conditions of wind damage, drought, and heat (Gora and Esquivel-Muelbert, 2021; Maia et al., 2020). These two mechanisms correspond to competition-induced carbon loss and drought-induced carbon loss, and they pose threats to smaller trees and larger trees separately. However, eCO2 also has the potential to promote tree survival by improving water use efficiency during drought (Brienen et al., 2017a, b; Van der Sleen et al., 2015). Liu et al. (2017) demonstrated, using simulations with a detailed soil–plant hydraulic model, that eCO2 mitigates drought risks by decreasing the fraction of days when the daily minimum xylem water potential is below a critical threshold. Findings from a global model by Yao et al. (2023) simulating hydraulics and demography also indicated that drought exposure could be alleviated under eCO2 in the Amazon. Besides, eCO2 effects are also regulated by hydroclimatic conditions. Fatichi et al. (2016) revealed that indirect effects on productivity from eCO2 tend to be more pronounced in water-limited ecosystems, although severe water stress can offset the expected CO2 fertilization effects (Kolby Smith et al., 2016). The magnitude of the water-saving effect is also modulated by the intensity and duration of water stress events (Birami et al., 2020). Therefore, given the interplay of enhanced photosynthesis, heightened competition, vulnerability due to larger size, and mitigating effects from water-saving benefits, the impact of eCO2 on carbon balance is not a straightforward monotonic relationship. The relative rates at which gross carbon fluxes change with eCO2 play a crucial role in determining the net changes in aboveground biomass (AGB) (Fig. 1).
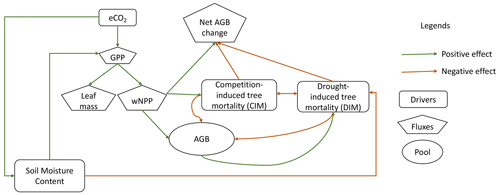
Figure 1A schematic plot illustrating the influence of eCO2 on forest biomass carbon balance. A positive effect means that the impacted variable is expected to increase under eCO2 compared to pre-industrial CO2. A positive effect on net AGB change means that eCO2 leads to a higher net carbon gain for AGB. When CO2 concentration rises, water use efficiency increases due to partial closure of stomata. Consequently, soil water consumption decreases, leading to an increase in soil moisture content (positive effect on soil moisture content (SMC)). This increase in SMC offers a degree of mitigation against drought exposure, known as water-saving benefits (negative effect on drought-induced mortality (DIM)). Simultaneously, eCO2 enhances photosynthesis, resulting in greater carbon gains (positive effect on canopy leaf mass and woody net primary production (wNPP)). This enhanced tree growth also intensifies competition, leading to natural self-thinning (positive effect on competition-induced mortality (CIM)). While a reduction in drought exposure through higher SMC offers a reduction in DIM, the presence of “more carbon available to lose” under eCO2 contributes to increased drought-induced biomass carbon loss due to drought mortality (negative effect of DIM on AGB). The combined effects of enhanced carbon gain, reduced drought exposure, and higher biomass density can influence net biomass carbon change either positively or negatively. GPP: gross primary productivity.
Given the unavailability of free-air CO2 enrichment (FACE) experiments and the scarcity of in situ measurements within the complex Amazon rainforest, employing process-based modeling emerges as a promising approach for investigating how eCO2 influences the accumulation and loss of biomass. Koch et al. (2021) demonstrated that models participating in Coupled Model Intercomparison Project 5 (CMIP5) and CMIP6 could reproduce the response of carbon gains attributed to tree growth in response to environmental drivers but were rather unable to reproduce the carbon losses observed in inventory data. This model shortcoming primarily results from the fact that CMIP5- and CMIP6-participating models do not include processes related to tree competition and that most of them ignore climate-induced mortality processes, although a new generation of global models are under development to address these research gaps (Eller et al., 2020; Koven et al., 2020; Yao et al., 2022). Yu et al. (2022) underscored this issue and showed that biomass loss due to the tree mortality was overestimated in dynamic global vegetation models (DGVMs) when compared to historical forest inventory measurements and that incorporating observation-based constraints into these models would lead to a reduction in carbon sink predictions by the end of the century. Therefore, conducting modeling studies with realistic representations of tree mortality and incorporating observation-based constraints are crucial steps toward achieving a more reliable projection of the evolution of carbon stocks in intact Amazon forests.
As the representation of mortality in most process-based models is based on prescribing a fixed-loss fraction of standing biomass stocks (Adams et al., 2013), there is a clear need for a more realistic simulation of tree-mortality-induced carbon loss. In Yao et al. (2023), an empirical hydraulic-failure module and a light-competition tree mortality module in the ORCHIDEE (Organising Carbon and Hydrology In Dynamic Ecosystems) land surface model were tested over the Amazon rainforest. This model has been calibrated to reproduce tree-size-dependent mortality rates at a site in Caxiuanã (a long-term throughfall exclusion experiment) and has proven effective in reproducing the increasing carbon loss due to tree mortality rate and the resulting basin-scale deceleration in the net carbon sink, observed in inventories from Hubau et al. (2020).
In this study, we explore the impact of eCO2 on forest growth, tree mortality, and drought recovery in the Amazon rainforest. Our analysis leverages the newly upgraded process-based model ORCHIDEE-CAN-NHA r7236 with competition- and drought-induced mortalities, following the methodology outlined in Yao et al. (2023). We conducted two factorial simulations – one with rising CO2 levels since 1901 and one without. The three key specific questions we address here are as follows. (i) Does eCO2 lead to a greater increase in tree mortality compared to productivity? (ii) Does eCO2 promote carbon loss more during wet years compared to dry years? (iii) Does eCO2 alleviate the impact of drought on net carbon balance of AGB and benefit drought recovery? Our hypothesis is that eCO2 leads to a smaller increase in tree mortality compared to carbon gain and that this net benefit for AGB changes is greater during dry years, contributing to accelerated drought recovery. The process-based model ORCHIDEE-CAN-NHA has been well calibrated. In brief, this model incorporates a mechanistic plant hydraulic architecture simulating water potentials at 30 min intervals within the soil–root–stem–leaf continuum. It also includes a drought-exposure-related tree mortality scheme and accounts for size-dependent tree mortality rates under exposure conditions. For a detailed model description, calibration information, and validation against observed datasets, please refer to Yao et al. (2022) and Sect. 2.
2.1 Model description
ORCHIDEE-CAN-NHA incorporates a plant hydraulic architecture that enables the modeling of water potential and hydraulic conductance along the vertical profile of plants. This module considers both vertical water flow driven by water potential gradients and the movement of water into or out of water storage pools, regulated by water capacitance. By simulating the plant hydrodynamics, we derive a critical indicator of plant water stress: the percentage loss of conductance (PLC). PLC has been demonstrated to correlate with tree mortality (Choat et al., 2012), e.g., ψ50, which represents the water potential at which 50 % of conductance is lost. Building on the simulation of ψ50, we have integrated an empirical tree mortality module that is based on drought exposure, which can help reproduce the size-dependent tree mortality pattern of higher tree mortality rates in cohorts with a larger circumference class. Within this framework, two crucial empirical parameters have been introduced: the drought exposure threshold and the fraction of tree mortality once this threshold is reached. These two parameters were calibrated using observed water potentials, sap flux transpiration, and stem mortality rates from a long-term throughfall exclusion experiment conducted at the Caxiuanã site located in the northeastern Amazon (Yao et al., 2022). The calibrated model has proven accurate in capturing the sensitivity of carbon fluxes to drought and the long-term trends in net carbon sink dynamics. This was demonstrated by comparing the simulated sensitivity of biomass loss rates and growth rates to water deficit against plot observations for the droughts of 2015 and 2010 (Yao et al., 2023). In addition to drought-induced tree mortality, ORCHIDEE also parametrizes the light-competition-induced self-thinning process (Joetzjer et al., 2022), which accounts for competition-induced tree mortality. The self-thinning process in ORCHIDEE is regulated by the quadratic mean diameter, where smaller trees are killed in order of priority.
As ORCHIDEE is a cohort-based model, we obtain woody carbon gain, woody carbon loss, and biomass carbon pools for 20 cohorts, associated with increasing circumference/diameter classes from small trees to large trees. Carbon gain in our model refers to wNPP, specifically that for cohorts with a diameter above 10 cm, aligning with inventory protocols. Carbon loss represents the amount of live biomass (with a diameter >10 cm) that is transferred to the woody litter pool due to tree mortality, resulting from events of continuous competition-induced mortality (killing small trees) and events regarding pulses of drought-induced mortality (killing large trees). Then we aggregate the grid-level carbon gain and carbon loss to the basin level, following the approach used by Brienen et al. (2015). Observational time series of carbon gains, losses, and the net carbon balance for Amazonian forests are obtained from Brienen et al. (2015). To gain a deeper insight into how eCO2 impacts carbon loss, we examined changes in both competition-induced tree mortality (self-thinning (CIM)) and drought-induced tree mortality (DIM) as distinct components. For drought mortality, we compared drought exposure under constant CO2 to assess how eCO2 alleviates the risk of tree mortality from hydraulic failure.
Following a TRENDY-type protocol (Seiler et al., 2022), we have implemented two distinct scenarios in our study. The first scenario maintains a constant CO2 concentration at the 1901 level while varying climate forcing (A1), whereas the second scenario permits variations in both CO2 concentration and climate forcing (A2). Here, A2 is similar to S2 from the TRENDY protocol despite the fact that we did not consider land cover change.
2.2 Climate-forcing data
The gridded climate-forcing dataset employed is CRUJRA v2.1 (Harris et al., 2020), used in the TRENDY simulations. CRUJRA v2.1 was created by re-gridding data taken from Japanese Reanalysis (JRA) data, a product of the Japanese Meteorological Agency. This re-gridded dataset was adjusted to align with monthly observation-based Climatic Research Unit (CRU) TS4.04 data (Harris et al., 2020). CRUJRA v2.1 provides 6 h meteorological variables from January 1901 to December 2019 at a spatial resolution of 0.5°.
2.3 Drought characteristics
Following Papastefanou et al. (2022), for the evaluation of drought area and severity, the maximum cumulative water deficit (MCWD) was used to compare droughts, as seen in Eqs. (1) and (2), with a fixed value for evapotranspiration (ET) of ∼100 mm per month being used (Phillips et al., 2009). When monthly rainfall (Pm) is below 100 mm, the forest undergoes a water deficit. This water deficit accumulates over the hydrological year from October in the previous year to September in the current one. The MCWD is the most negative value of the water deficit across all 12 months.
where m is the month from October to September.
Then the decadal mean of the MCWD over the entire period (μMCWD) was subtracted from the MCWD of a year with drought (MCWDi), giving an MCWD anomaly (Eq. 3).
We derived Z scores of MCWD time series at the annual scale by following Eq. (4) from Feldpausch et al. (2016), calculated according to
where σMCWD is the interannual standard deviation of the MCWD.
2.4 Drought resistance and resilience
For each drought event, drought resistance is defined as the change in the net biomass carbon sink during drought disturbance relative to its pre-drought state. A positive value indicates that drought conditions lead to an increase in the net carbon sink relative to non-stressed conditions, while negative values indicate a decrease in the net biomass carbon sink. A more negative value indicates higher vulnerability. Drought resilience refers to the ability of the net carbon sink to recover to the pre-drought state. It is computed as the difference in the net carbon sink between the post-drought period and the pre-drought state relative to the pre-drought period. Positive values indicate full recovery, where the net carbon sink after drought stress surpasses the pre-drought state, while negative values indicate incomplete recovery. A more negative ratio represents a more limited capacity for recovery. The calculations for drought resistance and resilience of net biomass carbon change followed Tao et al. (2022). We also used the net biomass carbon balance from 2 years before and 2 years after the drought event to represent pre- and post-drought forest conditions, respectively (Tao et al., 2022). Resistance and resilience were calculated for each pixel and for all the drought events during the past 4 decades, following Eqs. (1) and (2), and were reported at the basin scale by taking the median value across drought-affected pixels (ZMCWD below −1).
Here, Ypre is the pre-drought value of net biomass carbon change (carbon gain (CG) minus carbon loss (CL)), Ypost is the post-drought value of net biomass carbon change, and Ye is the signal during the drought event for net biomass carbon change.
3.1 The mean carbon gains and carbon losses over the Amazon rainforest
Our model simulations over the past 4 decades, driven by varying CO2 and climate forcing, reveal that CG slightly surpasses CL at the basin scale, resulting in a positive net carbon balance (“Net” in Fig. 2a). When we separate years into wet and dry categories based on the basin-scale median of Z-transformed MCWD (ZMCWD>0 for wet years and ZMCWD<0 for dry years), we find a net carbon sink during wet years and a net carbon source during dry years. This pattern arises because CG is lower and CL is higher during dry years compared to during wet years and vice versa. During dry years, competition-induced carbon loss (CIM) is a bit lower compared to during wet years, and increased drought-induced tree mortality (DIM) leads to higher CL. Under constant CO2 concentration conditions (A1 scenario), both CG and CL decrease compared to the A2 scenario, with the reduction in CG being more pronounced, resulting in a much smaller net carbon sink than under eCO2 conditions (Fig. 2b). Carbon loss from CIM also decreases during dry years compared to during wet years, and its fraction in the total carbon loss becomes lower as increases in drought-induced tree dieback can suppress self-thinning. When comparing the model simulations with and without eCO2, higher ΔCG during dry years compared to during wet years suggests the CO2 fertilization effect is more efficient during dry years (Fig. 2c). ΔCL is primarily affected by CIM, even though ΔCIM and ΔDIM have opposing effects on it (Fig. 2c). Our model simulation thus implies that the CO2 fertilization effect plays a dominant role in augmenting aboveground forest productivity (carbon gains) and (to a lesser extent) biomass loss rates from mortality. Our estimate falls within the upper range of the trend distribution, which is consistent with existing studies on the effects of eCO2, including those employing process-based models, analytical solutions, and ecological optimality theory (Table S1 in the Supplement).
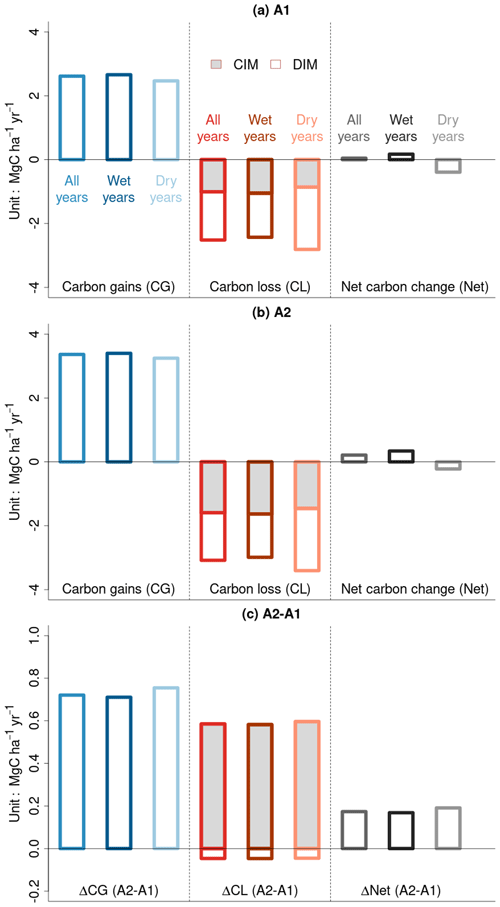
Figure 2Basin-scale averages of forest biomass carbon gains (CG), forest biomass carbon losses (CL), and the net balance of gains minus losses (Net) over the last 4 decades. (a) eCO2. (b) Constant CO2 at pre-industrial levels. (c) Difference between eCO2 and constant CO2 (Δ). By convention, gains are positive and losses are negative in (a) and (b). In panel (c), ΔCL is calculated as the difference between the absolute values of CL in panels (a) and (b). Dry years are defined as those in which the median of the Z-transformed cumulative water deficit (ZMCWD) at the basin scale falls below 0. For biomass carbon loss, CIM represents competition-induced self-thinning processes, and DIM represents drought-induced tree mortality processes.
3.2 Effect of eCO2 on the trends of the net biomass carbon sink, carbon gains, and carbon losses
Our A2 simulation, which accounts for varying climate and CO2 concentration, shows a decline in the net biomass carbon sink since 1980, decreasing at a rate of 0.006 MgC ha−1 yr−2 (6 kgC ha−1 yr−2). This decelerating trend can be predominantly attributed to the increase in carbon loss resulting from tree mortality, which amounts to 0.014 MgC ha−1 yr−2, surpassing the enhanced carbon gain trend of 0.008 MgC ha−1 yr−2. The trend of the biomass sink in the A2 scenario has the same sign as that in forest inventory records, but it is 60 % smaller in magnitude (see Fig. 3). When CO2 concentration is held constant, the A1 scenario indicates a larger decline in the net carbon sink. This more negative trend is primarily driven by reduced carbon gains, while carbon loss increases less.
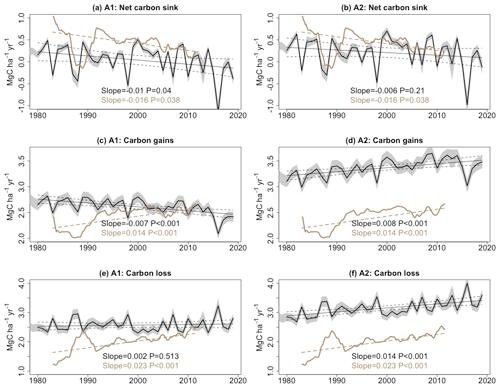
Figure 3Trends in (a, b) the net carbon sink for aboveground live biomass, (c, d) carbon gains, and (e, f) carbon losses from tree mortality; these were obtained from ORCHIDEE model simulations (black lines) and forest inventory data (brown lines). Shading represents the 95 % confidence interval. The slopes and associated P values are from linear regression models. It should be noted that the number of measurements for each year in the inventory varies and a “linear mixed-effects model” was used to account for the weight associated with different sampling plot areas and their monitoring time lengths. Therefore, in the inventory-pooling results, the trend of the net carbon sink is not equal to the difference between trends in carbon gain and carbon loss.
3.3 Effect of eCO2 on competition-induced and drought-induced carbon losses
As described in Sect. 3.1, carbon loss resulting from tree mortality can be categorized into two distinct processes: competition-induced mortality (CIM) and drought-induced mortality (DIM). Figure 4 illustrates the trends in carbon loss attributed to these two processes. In the A2 scenario, simulated CIM displays no significant trend (slope =0.001 MgC ha−1 yr−2; P=0.738). However, when CO2 remains constant in the A1 scenario, this term exhibits a notable decrease (slope MgC ha−1 yr−2; P<0.001). In contrast, both the A1 and A2 scenarios exhibit significant increasing trends in DIM (slope =0.013 MgC ha−1 yr−2; P<0.05). Consequently, the lack of a significant overall trend in total carbon loss in the A1 scenario can be attributed to the opposing effects of CIM and DIM. Our model simulations reaffirm that the increasing carbon loss in the A2 scenario is primarily a result of higher drought-induced tree mortality. Without the sustained increase in CO2, the net carbon sink would have diminished at a faster pace (slope MgC ha−1 yr−2 in the A1 scenario, and slope MgC ha−1 yr−2 in the A2 scenario).
3.4 Water conditions mediate biomass carbon flux responses to eCO2
The impact of eCO2 on biomass carbon sinks is influenced by prevailing water conditions. To explore how hydroclimatic conditions regulate the impact of eCO2 on carbon gain and carbon loss, we focused on three recent megadrought events (2005, 2010, and 2015/2016). In line with the methodology applied by Pan et al. (2022) to eCO2 vs. control experiments, we used the ratio between the enhanced CO2 scenario (A2) and the constant CO2 scenario (A1) to assess the relative response (R) of the ecosystem level to carbon gain (RCG) and carbon loss (RCL). During these three drought events, we found that forests in drier climate zones (a more negative MCWD) exhibited greater RCG compared to their wetter counterparts (Fig. 5), and this model response prevails across all cohorts, with larger-sized cohorts showing lower RCG and less negative sensitivity of RCG to the MCWD due to more carbon allocation to smaller cohorts (Fig. S1 in the Supplement). Interestingly, RCL does not show a monotonic change from small to large cohorts, even though the average over the drought epicenter indicates higher RCL in smaller cohorts (Fig. S2). Self-thinning may not always occur due to suppression by DIM. Therefore, even though higher RCG is found under eCO2 along the water stress gradient, self-thinning does not always change coordinately. Here, RCL is mainly contributed by drought-induced carbon loss (RCL_DIM), where self-thinning is suppressed. However, RCL_DIM does not exhibit a significant correlation with the MCWD (Fig. 5). It is worth noting that DIM-induced carbon loss is influenced by two key factors: background biomass density and tree mortality rate. The former one is boosted by eCO2, indicating “more carbon available to lose” (Fig. 5), while the response of the latter is the opposite because eCO2 leads to a reduction in stomatal conductance and transpiration, alleviating water stress, which is indicated by fewer drought exposure days (Fig. S3) and a lower fraction of trees killed due to DIM in the A2 scenario than in the A1 scenario across most regions within the epicenter of the drought events (; Fig. 5). This suggests that eCO2 has a positive impact on mitigating the effects of drought on biomass loss driven by DIM.
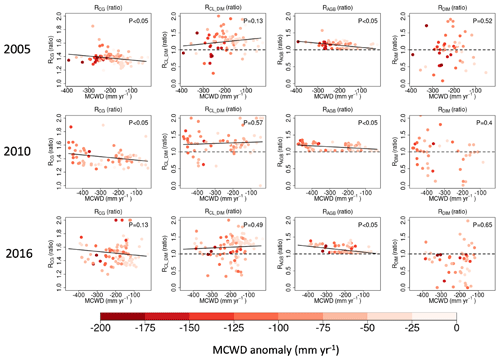
Figure 5The effect of eCO2 on carbon gain (RCG), carbon loss due to drought-induced tree mortality (RCL_DIM), AGB (RAGB), and the proportion of trees affected by DIM (RDIM) in relation to water conditions and drought intensity in the years 2005, 2010, and 2016. Water conditions are characterized by the MCWD during the drought year (on the horizontal axis), where negative values indicate a drier climate. Dots are color-coded to reflect the drought intensity characterized by the MCWD anomaly, with darker colors indicating more severe water deficits. The dots shown in the panels correspond to pixels located in the epicenter of the drought, featuring ZMCWD values below −1. This threshold is set to ensure an adequate number of pixels for our analysis.
3.5 Effect of eCO2 on drought recovery
After a drought, eCO2 supports faster or more complete recovery due to growth enhancement (De Kauwe et al., 2021). eCO2 can also enable deeper root depth and thus better access to deeper soil moisture (Iversen, 2010). In addition to investigating the impact of eCO2 on carbon loss during droughts, our study delved into whether eCO2 confers benefits to drought recovery, considering the likely promoted growth and two different tree mortality regimes of CIM and DIM. To identify past drought events, we calculated the MCWD for each year and each pixel. Droughts were defined as pixels in a year with an MCWD Z score falling below −1 since 1980. This threshold, while not extremely severe, was chosen to ensure enough pixels for analysis. Following the methodology outlined by Tao et al. (2022), we aggregated the pixel-level results to the basin scale by calculating the median value across all pixels. Considering the findings of Tao et al. (2022), which indicated increased drought resistance across a gradient of drought severity using C-band radar signals, we examined whether the process-based model could reproduce this drought response. Figure 6 illustrates the relationship between drought resistance and resilience of net biomass carbon change in relation to drought severity, expressed as ZMCWD. In both the A1 and A2 scenarios, we observed a well-expected declining trend in resistance as drought severity increased. When drought severity was defined using the absolute value of the MCWD anomaly, a similar declining trend of resistance for net carbon change was found (Fig. S4). Notably, the rainforests did not fully recover to their pre-drought conditions following drought events of a higher severity – in the A1 scenario, 62±12 % of the area located in the drought epicenter showed resilience below 0, whereas in the A2 scenario, this was 59±16 %. When examining the differences in drought resistance and resilience between the A1 and A2 scenarios, our findings indicate that eCO2 enhances both drought resistance and resilience. For drought-sensitive areas where the tree mortality regime shifts from CIM to DIM, their drought resistance and resilience are lower than in insensitive areas due to higher carbon loss. This suggests that elevated CO2 levels contribute to improved forest resilience and enhance the ability of forests to withstand and recover from drought events.
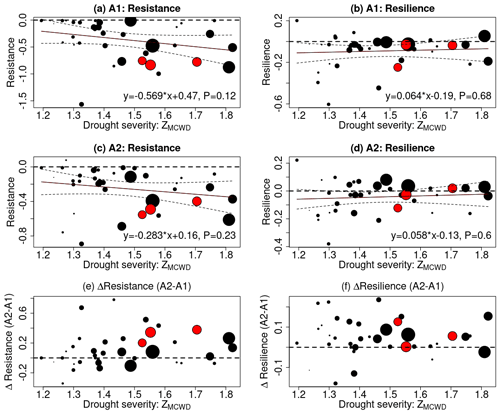
Figure 6The effect of eCO2 on drought recovery in terms of resistance and resilience of net biomass carbon change. For each drought event, the median resistance, resilience, and drought severity of the drought-affected pixels (ZMCWD below −1) were calculated (shown as dots in each panel). Drought severity was defined by the ZMCWD. The size of the dots indicates the area within the drought epicenter. The dots for 2005, 2010, and 2016 are color-coded in red. A trend fit was applied to panels (a)–(d), with its equation labeled and 95 % confidence interval marked by dashed lines. Resistance was computed, where lower values mean a more pronounced reduction in the net biomass carbon sink during a drought. Resilience was also computed, where positive values indicate complete recovery of the forest to pre-drought conditions, while negative values signify incomplete recovery. In panels (a)–(d), a horizontal dashed line indicates resistance and resilience values of zero. In panels (e)–(f), a horizontal dashed line was drawn to denote no change in resistance or resilience under eCO2 conditions.
The CO2 fertilization effect has gained widespread recognition as a primary driver of vegetation greening, observed as an increase in leaf area index (LAI) in most regions around the world (Zhu et al., 2016). Our model, forced by eCO2, also captures LAI greening in most areas over the basin, while a constant CO2 setup results in browning (Fig. S5). However, it is important to clarify that this greening phenomenon does not necessarily translate into increased biomass accumulation because the carbon allocation shift and processes that control biomass loss can be decoupled from the increasing trend of LAI and productivity (Fan et al., 2023). The dynamics of the net carbon sink are fundamentally shaped by the balance between productivity and carbon loss. The concept of a growth–mortality trade-off, or high gain/high loss, has been observed across spatial gradients (Wright et al., 2010), but it remains more difficult to prove in terms of expressed temporal changes as “faster gains imply higher losses”. This is because one can observe an acceleration in both gains and losses, as noted by Hubau et al. (2020), but a causal relation between the two cannot be empirically proven. A demography model including more detailed stand dynamics could help test this hypothesis. eCO2 enhancing tree competition in wet tropical forests has the potential to couple faster gains with higher losses and thus may not universally lead to a boost in the net carbon sink due to an accelerated carbon turnover rate (Walker et al., 2021). Future CO2 fertilization could potentially increase not only recruitment and growth but also tree mortality (McDowell et al., 2020). In our study, we conducted model experiments to disentangle the effect of eCO2 on forest biomass carbon fluxes, including carbon gain, carbon loss, and net carbon balance. Our model simulations revealed a compelling insight. When we deactivated the CO2 fertilization effect, the model simulated an LAI browning trend (Fig. S5) and a declining trend in carbon gain compared to forest inventory observations (Fig. 2). These findings align with a similar compensatory effect on LAI from rising CO2 in TRENDY models for tropical forests, as noted by Winkler et al. (2021). Our results also underscored that eCO2 not only drives enhanced biomass carbon gains but also plays a pivotal role in shaping carbon losses (Figs. 3 and 4). Specifically, turning off the eCO2 effect leads to a dampened increase in carbon loss. This attenuation is primarily attributable to the muted response of natural self-thinning-related tree mortality, which is determined by less growth (i.e., the deceleration of carbon gain). Meanwhile, the more negative net carbon sink trend observed in the A1 scenario is primarily driven by the deceleration of carbon gain. The basin-scale average shows that CL_DIM (carbon loss driven by drought-induced tree mortality) is lower under eCO2 but that CL_ST (carbon loss driven by self-thinning) is highly promoted under eCO2. Thus, ΔCL_DIM and ΔCL_ST oppose each other, although ΔCL_ST dominates the magnitude of ΔCL (Fig. 1c). Such net forest biomass carbon loss in the absence of eCO2 was similar to the findings of de Almeida Castanho et al. (2016), in which multiple model simulations were used. They showed a decline in biomass over the past several decades when not considering eCO2 effects, although tree mortality had not been incorporated yet. Our work advances this understanding by separating the contributions of competition-induced and drought-induced tree carbon loss and providing evidence that eCO2 benefits the net forest biomass carbon sink over the Amazon rainforest, although such benefit may still not be sufficient to offset the carbon loss caused by prolonged external climate stressors, such as long-term temperature-induced carbon loss (Sullivan et al., 2020).
The response of biomass carbon flux dynamics to eCO2 is intricately linked to water availability. Taking the years 2005, 2010, and 2016 as examples, the enhancement ratio of carbon gain (RCG) exhibits a negative correlation with the water deficit metric of the MCWD, indicating that the eCO2 effect is more pronounced in drier regions. Pan et al. (2022) demonstrated that extra-tropical woody ecosystems characterized by drier baseline climates tend to exhibit a higher average enhancement in aboveground carbon gain in response to eCO2. Our model simulations suggest that a similar pattern may persist in wet tropical forests within the Amazon as well. The negative interactions found between eCO2 and water deficit suggest that vegetation in drier climates can benefit more from the combination of enhanced photosynthesis, reduced photorespiration, and increased WUE. Alongside potential growth advantages, the tree-ring-width data analysis by Zuidema et al. (2020) reveals that eCO2-induced partial stomata closure and reduced transpiration may attenuate the cooling effect on leaf surfaces, potentially pushing leaf surface temperatures beyond the optimal range for photosynthesis. Our modeling results, showing enhanced carbon gain over the Amazon rainforest, suggest that temperature may not have exceeded the optimal range in this case, given the mean annual land surface temperature under eCO2 increases by around 0.26 °C at most during the 2015–2016 El Niño (Fig. S6). Nonetheless, the potential shift in sensitivity within the carbon flux response of tropical forests to eCO2, depending on the balance between benefits and potential temperature-related challenges, emphasizes the necessity for optimizing and refining the process-based representation of climate–growth relationships. For instance, employing various sensitivity scenarios involving temperature intervals and CO2 concentrations can offer valuable insights for identifying the critical threshold beyond which the benefits of eCO2 do not outweigh the adverse high-temperature effects.
While self-thinning-induced carbon loss is heightened in the eCO2 scenario because of increased competition, drought-induced carbon loss might not always be exacerbated under eCO2, although there is more biomass built up over time that is available for carbon loss, revealing the influence of water-saving conditions on carbon loss. An important indicator we employed in our model, cumulative drought exposure, reveals a reduction over most areas when accounting for the water-saving effects driven by eCO2 (Fig. S3). Regarding tree mortality triggered by drought events, our modeling work confirms alleviated drought exposure, which is consistent with previous modeling findings that eCO2 brings about water saving due to increased WUE, thus enhancing vegetation productivity and decreasing the probability of forest dieback in the eastern Amazon basin being threatened by drier and warmer climate scenarios (Huntingford et al., 2013; Lapola et al., 2009; Zhang et al., 2015); however, these studies mainly considered enhanced productivity with respect to sustaining the biomass rather than carbon loss changes. The strength of this mitigation effect depends on the intensity and duration of stress (Lapola et al., 2009) and could postpone the point at which a forest shifts from being a carbon sink to a carbon source (Feng et al., 2018). However, the decreased frequency of tree mortality risk, combined with an increase in background biomass stock facilitated by eCO2, contributes to uncertainty regarding the fate of carbon loss.
In our modeling study, we found an increase in both WUE and tree growth (Figs. 1 and S7). While van der Sleen et al. (2015) reported no growth stimulation of tropical trees by means of CO2 fertilization, they did find an increase in WUE in their tree-ring-width analysis, which focused on a fixed tree size class. However, it has been argued by Brienen et al. (2017b) that this approach, aimed at removing the effect of tree size, might lead to a biased interpretation of growth trends, particularly when there is a clustered age distribution with fast-growing and slow-growing trees coexisting. Concerning the less controversial increase in WUE, Brienen et al. (2017a) suggested that the observed trends could be related to developmental effects rather than solely being the result of climate and CO2 effects on WUE. It is crucial to investigate and distinguish these factors as WUE varies with tree development stages, especially in broadleaf forests. Partial stomatal closure, driven by increasing constraints in water transport with tree height and increasing photosynthesis due to greater light availability with tree height, can lead to changes in intrinsic WUE (McDowell et al., 2011). To better isolate the effects of external factors, such as eCO2, through size-stratified sampling and account for the varying tree gas regulation strategies throughout a tree's lifespan, it is essential to incorporate a stratified simulation of stomatal conductance as well as corresponding photosynthesis. While our ORCHIDEE model features a stratified LAI pattern, it does not yet include stratified simulation of a stomatal conductance. Implementing a more detailed water budget per canopy layer would provide a more comprehensive understanding of tree-height-related shifts in WUE.
Regarding drought resistance and resilience, C-band radar data have demonstrated a decrease in resistance to drought over the Amazon rainforest during the past 3 decades, while forest resilience has not declined significantly (Tao et al., 2022). Our model simulation also detected the well-expected phenomenon of decreased resistance with increasing drought severity. The resistance and resilience of net biomass carbon balance were found to benefit from eCO2, which is broadly consistent with the enhanced resistance to drought due to restricted stomatal conductance and improved WUE observed in Feng et al. (2018). This suggests that eCO2 can enhance the recovery of ecosystem carbon uptake after short-term drought events.
Several uncertainties warrant in-depth investigations. Whether eCO2 would lead to biomass growth also depends on the carbon allocation strategies and, subsequently, the carbon turnover rate (Friend et al., 2014; Hofhansl et al., 2016), which has been found to be governed by hydraulic constraints, such as the hydraulic adjustment of the ratio between leaf area and sapwood area (Trugman et al., 2019). Given that both tree productivity and mortality responses during drought are sensitive to hydraulic traits (Anderegg et al., 2016, 2018), incorporating varying hydraulic traits that are adaptive to the environment will be highly important (Madani et al., 2018). For example, tree mortality risk is intricately linked to plant water use strategies, with isohydric tree species exhibiting a lower xylem embolism risk due to their tendency to close stomata earlier to conserve water. In contrast, anisohydric tree species, characterized by less conservative water use strategies, may derive more significant benefits from eCO2-induced partial stomatal closure. Additionally, the analysis of water deficit affiliation has indicated that genera affiliated with wetter climate regimes exhibit a higher risk of drought-induced tree mortality (Esquivel-Muelbert et al., 2017). Exploring the interactions between eCO2 and varying hydraulic vulnerabilities would be a potential avenue for further examining the effects of eCO2 on biomass carbon dynamics. Besides hydraulic-failure-induced tree mortality, other possible sources, including carbon starvation, should be included as more carbon gain enhanced by eCO2 would delay the depletion of carbohydrate reserves. Furthermore, it is essential to consider the legacy effects of drought, a dimension that has not been addressed in process-based modeling. Yang et al. (2023) used a first-order kinetics model to account for the gradual decomposition of coarse woody debris, yielding a better correspondence between net biomass carbon change and variability in the atmospheric CO2 growth rate. The legacy effects from tree mortality should be carefully revisited, given the evidence suggesting that external drivers can lead to increased mortality for at least 2 years after a climatic event (Aleixo et al., 2019). Regarding the strength and persistence of eCO2, previous studies have suggested that such fertilization effects could slow down (Peñuelas et al., 2017), and the eCO2 effect has declined in recent years, possibly due to nutrient limitation (Winkler et al., 2021). Wieder et al. (2015) demonstrated that accounting for nitrogen and nitrogen–phosphorus limitation lowers the projected productivity and could even turn terrestrial ecosystems into carbon sources. Fleischer et al. (2019) highlighted the important role of phosphorus acquisition and use strategies in regulating the forest response to eCO2, effectively reducing the expected stimulation by 50 % over the Amazon rainforest. The lack of downregulation on fertilization in the model could lead to an overestimation of eCO2 effects. In addition to the absence of downregulation due to nutrient availability, uncertainties in carbon allocation could also contribute to differences in baseline values compared to inventory data. In the ORCHIDEE model, carbon allocation among biomass components adheres to the “pipe model” theory, which dictates the relationship between leaf area, sapwood area, and fine-root area (Sitch et al., 2003). However, the carbon allocation process remains relatively unconstrained and requires further observation data for benchmarking purposes. Given that nutrient availability influences productivity and adjustments in carbon allocation, a nutrient-enabled version of the model would help elucidate ecosystem responses to eCO2. Therefore, estimating the strength and persistence of the CO2 fertilization effect under future climate scenarios remains challenging (Nölte et al., 2023). Additional observations are imperative, and the Amazon free-air CO2 enrichment (AmazonFACE) project will be a strong observational constraint on our knowledge of the rainforest response to eCO2 (Lapola and Norby, 2014). We have also provided estimates of carbon gain and carbon loss in response to the planned CO2 increase (i.e., 200 ppm above ambient levels) for this forest for the period from 2010 to 2020. Our simulations indicate an enhancement in gross primary productivity (GPP) of ∼34 % and an enhancement in wNPP of ∼55 % (diameter at breast height (DBH) >10 cm) throughout the simulation period. These values are higher compared to those from simulations conducted with nutrient-cycle-enabled models, as reported by Fleischer et al. (2019). Obtaining more experimental data to illustrate the interactions between water and nutrient availability and their impacts on the CO2 fertilization effect would aid in constraining model responses, thus enabling more accurate predictions of the Amazon rainforest's response to future climate change.
In our study, we conducted offline simulations and found that eCO2 leads to an increase in WUE (Fig. S7), which could partially mitigate drought risk through soil–atmosphere feedback mechanisms. However, it is noteworthy that CO2-induced physiological effects reduce ET (evapotranspiration) and, subsequently, precipitation in a land–atmosphere coupled mode. A recent study using coupled climate model simulations has highlighted that the reduction in ET under eCO2 and its impact on precipitation contribute to potential water stress (Li et al., 2023; Skinner et al., 2018). Tree dieback indeed leads to a reduction in plant transpiration and also decreases the soil moisture consumption. We found that eCO2 leads to an increase in land surface temperature of 0–0.26 °C, based on the simulation during the 2015–2016 El Niño (Fig. S6). Therefore, given the contribution of moisture recycling to precipitation over the Amazon rainforest, a comprehensive investigation into the effects of eCO2 on biomass carbon dynamics, such as whether eCO2 can mitigate the negative effects of water stress due to changes in precipitation, should be conducted in a coupled mode, including a tree mortality module to capture the intricate interactions among these components.
In summary, this work offers a comprehensive quantitative assessment at the basin scale of how eCO2 influences aboveground biomass carbon gain and carbon loss in a warming and increasingly water-stressed climate. We systematically disentangle the effect of eCO2 in this complex ecosystem. Our findings not only underscore the role of eCO2 in shaping the high gain/high loss pattern but also highlight its water-saving benefits. Additionally, we identify an enhancement in drought resistance and resilience attributed to eCO2 as it accelerates drought recovery. Our improved model, which separates tree mortality schemes into competition-driven and drought-driven mechanisms, offers a more comprehensive understanding of carbon fluxes in response to eCO2, a perspective that cannot be solely attained through field experiments. With the likelihood of more frequent and intense drought events in the near future, these findings serve as a compelling impetus for further modeling and observational efforts aimed at gaining deeper insights into the role of eCO2 in predicting the forest biomass carbon budget and ecosystem vulnerability within the Amazon rainforest.
The ORCHIDEE-CAN-NHA r7236 model code used in this study is deposited at https://forge.ipsl.jussieu.fr/orchidee/browser/branches/publications/ORCHIDEE_CAN_NHA (last access: 17 June 2021) and archived at https://doi.org/10.14768/8C2D06FB-0020-4BC5-A831-C876F5FBBFE9 (Yao, 2021).
CRUJRA v2.1 climate-forcing data can be found at https://catalogue.ceda.ac.uk/uuid/10d2c73e5a7d46f4ada08b0a26302ef7 (Harris, 2020). The forest inventory dataset can be found at https://doi.org/10.5521/ForestPlots.net/2014_4 (Brienen et al., 2014).
The supplement related to this article is available online at: https://doi.org/10.5194/esd-15-763-2024-supplement.
PC and YY designed the study. YY ran the simulation, analyzed the outputs, and drafted the paper. All authors contributed to the final paper.
The contact author has declared that none of the authors has any competing interests.
Publisher’s note: Copernicus Publications remains neutral with regard to jurisdictional claims made in the text, published maps, institutional affiliations, or any other geographical representation in this paper. While Copernicus Publications makes every effort to include appropriate place names, the final responsibility lies with the authors.
This work was financially supported by the CLAND Convergence Institute, funded by the ANR (grant no. 16-CONV-0003). This study was also supported by the CALIPSO (Carbon Loss In Plants, Soils and Oceans) project, funded through the generosity of Eric and Wendy Schmidt on the recommendation of the Schmidt Science Fellows program. Yitong Yao also acknowledges support from a Make Our Planet Great Again (MOPGA) scholarship.
This research received support through Schmidt Sciences, LLC. This work was also financially supported by the CLAND Convergence Institute, funded by the ANR (grant no. 16-CONV-0003).
This paper was edited by Anping Chen and reviewed by two anonymous referees.
Adams, H. D., Williams, A. P., Xu, C., Rauscher, S. A., Jiang, X., and McDowell, N. G.: Empirical and process-based approaches to climate-induced forest mortality models, Front. Plant Sci., 4, 438, https://doi.org/10.3389/fpls.2013.00438, 2013.
Aleixo, I., Norris, D., Hemerik, L., Barbosa, A., Prata, E., Costa, F., and Poorter, L.: Amazonian rainforest tree mortality driven by climate and functional traits, Nat. Clim. Change, 9, 384–388, 2019.
Anderegg, W. R., Klein, T., Bartlett, M., Sack, L., Pellegrini, A. F., Choat, B., and Jansen, S.: Meta-analysis reveals that hydraulic traits explain cross-species patterns of drought-induced tree mortality across the globe, P. Natl. Acad. Sci. USA, 113, 5024–5029, 2016.
Anderegg, W. R., Konings, A. G., Trugman, A. T., Yu, K., Bowling, D. R., Gabbitas, R., Karp, D. S., Pacala, S., Sperry, J. S., and Sulman, B. N.: Hydraulic diversity of forests regulates ecosystem resilience during drought, Nature, 561, 538–541, 2018.
Birami, B., Nägele, T., Gattmann, M., Preisler, Y., Gast, A., Arneth, A., and Ruehr, N. K.: Hot drought reduces the effects of elevated CO2 on tree water-use efficiency and carbon metabolism. New Phytol., 226, 1607–1621, 2020.
Boulton, C. A., Lenton, T. M., and Boers, N.: Pronounced loss of Amazon rainforest resilience since the early 2000s, Nat. Clim. Change, 12, 271–278, 2022.
Brienen, R. J. W., Phillips, O. L., Feldpausch, T. R., Gloor, E., Baker, T. R., Lloyd, J., Lopez-Gonzalez, G., Monteagudo-Mendoza, A., Malhi, Y., Lewis, S. L., Vásquez Martinez, R., Alexiades, M., Álvarez Dávila, E., Alvarez-Loayza, P., Andrade, A., Aragão, L. E. O. C., Araujo-Murakami, A., Arets,E. J. M. M., Arroyo, L., Aymard, G. A., Baraloto, C., Barroso, J., Bonal, D., Boot, R. G. A., Camargo, J. L., Castilho, C. V., Chama, V., Chao, K. J., Chave, J., Comiskey, J. A., Cornejo Valverde, F., da Costa, L., Oliveira, E. A., Di Fiore, A., Erwin, T. L., Fauset, S., Forsthofer, M., Grahame, E. S., Groot, N., Hérault, B., Higuchi, N., Honorio, E. C., Keeling, H., Killeen, T. J., Laurance, W. F., Laurance, S., Licona, J., Magnusson, W. E., Marimon, B. S., Marimon-Junior, B. H., Mendoza, C., Neill, D. A., Nogueira, E. M., Núñez, P., Pallaqui Camacho, N. C., Parada, A., Pardo, G., Peacock, J., Peña-Claros, M., Pickavance, G. C., Pitman, N. C. A., Poorter, L., Prieto, A., Quesada, C. A., Ramírez, F., Ramírez-Angulo, H., Restrepo, Z., Roopsind, A., Rudas, A., Salomão, R. P., Schwarz, M., Silva, N., Silva-Espejo, J. E., Silveira, M., Stropp, J., Talbot, J., ter Steege, H., Teran-Aguilar, J., Terborgh, J., Thomas-Caesar, R., Toledo, M., Torello-Raventos, M., Umetsu, R. K., van der Heijden, G. M. F., van der Hout, P., Guimarães Vieira, I. C., Vieira, S. A., Vilanova, E., Vos, V., and Zagt, R. J.: Plot Data from: Long-term decline of the Amazon carbon sink, ForestPlots.NET [data set], https://doi.org/10.5521/ForestPlots.net/2014_4, 2014.
Brienen, R. J., Phillips, O. L., Feldpausch, T. R., Gloor, E., Baker, T. R., Lloyd, J., Lopez-Gonzalez, G., Monteagudo-Mendoza, A., Malhi, Y., and Lewis, S. L.: Long-term decline of the Amazon carbon sink, Nature, 519, 344–348, 2015.
Brienen, R., Gloor, E., Clerici, S., Newton, R., Arppe, L., Boom, A., Bottrell, S., Callaghan, M., Heaton, T., and Helama, S.: Tree height strongly affects estimates of water-use efficiency responses to climate and CO2 using isotopes, Nat. Commun., 8, 288, https://doi.org/10.1038/s41467-017-00225-z, 2017a.
Brienen, R. J., Gloor, M., and Ziv, G.: Tree demography dominates long-term growth trends inferred from tree rings, Glob. Change Biol., 23, 474–484, 2017b.
Choat, B., Jansen, S., Brodribb, T. J., Cochard, H., Delzon, S., Bhaskar, R., Bucci, S. J., Feild, T. S., Gleason, S. M., and Hacke, U. G.: Global convergence in the vulnerability of forests to drought, Nature, 491, 752–755, 2012.
de Almeida Castanho, A. D., Galbraith, D., Zhang, K., et al. Changing Amazon biomass and the role of atmospheric CO2 concentration, climate, and land use, Global Biogeochem. Cy., 30, 18–39, 2016.
De Kauwe, M. G., Medlyn, B. E., and Tissue, D. T.: To what extent can rising [CO2] ameliorate plant drought stress?, New Phytol., 231, 2118–2124, 2021.
Eller, C. B., Rowland, L., Mencuccini, M., Rosas, T., Williams, K., Harper, A., Medlyn, B. E., Wagner, Y., Klein, T., and Teodoro, G. S.: Stomatal optimization based on xylem hydraulics (SOX) improves land surface model simulation of vegetation responses to climate, New Phytol., 226, 1622–1637, 2020.
Esquivel-Muelbert, A., Galbraith, D., Dexter, K. G., Baker, T. R., Lewis, S. L., Meir, P., Rowland, L., Costa, A. C. L. d., Nepstad, D., and Phillips, O. L.: Biogeographic distributions of neotropical trees reflect their directly measured drought tolerances, Sci. Rep., 7, 8334, https://doi.org/10.1038/s41598-017-08105-8, 2017.
Fan, L., Wigneron, J.-P., Ciais, P., Chave, J., Brandt, M., Sitch, S., Yue, C., Bastos, A., Li, X., and Qin, Y.: Siberian carbon sink reduced by forest disturbances, Nat. Geosci., 16, 56–62, 2023.
Fatichi, S., Leuzinger, S., Paschalis, A., Langley, J. A., Donnellan Barraclough, A., and Hovenden, M. J.: Partitioning direct and indirect effects reveals the response of water-limited ecosystems to elevated CO2, P. Natl. Acad. Sci. USA, 113, 12757–12762, 2016.
Feldpausch, T., Phillips, O., Brienen, R., Gloor, E., Lloyd, J., Lopez-Gonzalez, G., Monteagudo‐Mendoza, A., Malhi, Y., Alarcón, A., and Álvarez Dávila, E.: Amazon forest response to repeated droughts, Global Biogeochem. Cy., 30, 964–982, 2016.
Feng, X., Uriarte, M., González, G., Reed, S., Thompson, J., Zimmerman, J. K., and Murphy, L.: Improving predictions of tropical forest response to climate change through integration of field studies and ecosystem modeling, Glob. Change Biol., 24, e213–e232, 2018.
Fleischer, K., Rammig, A., De Kauwe, M. G., Walker, A. P., Domingues, T. F., Fuchslueger, L., Garcia, S., Goll, D. S., Grandis, A., and Jiang, M.: Amazon forest response to CO2 fertilization dependent on plant phosphorus acquisition, Nat. Geosci., 12, 736–741, 2019.
Friend, A. D., Lucht, W., Rademacher, T. T., Keribin, R., Betts, R., Cadule, P., Ciais, P., Clark, D. B., Dankers, R., and Falloon, P. D.: Carbon residence time dominates uncertainty in terrestrial vegetation responses to future climate and atmospheric CO2, P. Natl. Acad. Sci. USA, 111, 3280–3285, 2014.
Gora, E. M. and Esquivel-Muelbert, A.: Implications of size-dependent tree mortality for tropical forest carbon dynamics, Nat. Plants, 7, 384–391, 2021.
Harris, I. C.: CRU JRA v2.1: A forcings dataset of gridded land surface blend of Climatic Research Unit (CRU) and Japanese reanalysis (JRA) data, Jan.1901–Dec.2019, Centre for Environmental Data Analysis, https://catalogue.ceda.ac.uk/uuid/10d2c73e5a7d46f4ada08b0a26302ef7, 2020.
Harris, I., Osborn, T. J., Jones, P., and Lister, D.: Version 4 of the CRU TS monthly high-resolution gridded multivariate climate dataset, Sci. Data, 7, 109, https://doi.org/10.1038/s41597-020-0453-3, 2020.
Hofhansl, F., Andersen, K. M., Fleischer, K., Fuchslueger, L., Rammig, A., Schaap, K. J., Valverde-Barrantes, O. J., and Lapola, D. M.: Amazon forest ecosystem responses to elevated atmospheric CO2 and alterations in nutrient availability: filling the gaps with model-experiment integration, Front. Earth Sci., 4, 19, https://doi.org/10.3389/feart.2016.00019, 2016.
Hubau, W., Lewis, S. L., Phillips, O. L., Affum-Baffoe, K., Beeckman, H., Cuní-Sanchez, A., Daniels, A. K., Ewango, C. E., Fauset, S., and Mukinzi, J. M.: Asynchronous carbon sink saturation in African and Amazonian tropical forests, Nature, 579, 80–87, 2020.
Huntingford, C., Zelazowski, P., Galbraith, D., Mercado, L. M., Sitch, S., Fisher, R., Lomas, M., Walker, A. P., Jones, C. D., and Booth, B. B.: M. Simulated resilience of tropical rainforests to CO2-induced climate change, Nat. Geosci., 6, 268–273, 2013.
Iversen, C. M. Digging deeper: fine-root responses to rising atmospheric CO2 concentration in forested ecosystems, New Phytol., 186, 346–357, 2010.
Joetzjer, E., Maignan, F., Chave, J., Goll, D., Poulter, B., Barichivich, J., Maréchaux, I., Luyssaert, S., Guimberteau, M., and Naudts, K.: Effect of tree demography and flexible root water uptake for modeling the carbon and water cycles of Amazonia, Ecol. Model., 469, 109969, https://doi.org/10.1016/j.ecolmodel.2022.109969, 2022.
Koch, A., Hubau, W., and Lewis, S. L.: Earth system models are not capturing present-day tropical forest carbon dynamics, Earth's Future, 9, e2020EF001874, https://doi.org/10.1029/2020EF001874, 2021.
Kolby Smith, W., Reed, S. C., Cleveland, C. C., Ballantyne, A. P., Anderegg, W. R., Wieder, W. R., Liu, Y. Y., and Running, S. W.: Large divergence of satellite and Earth system model estimates of global terrestrial CO2 fertilization, Nat. Clim. Change, 6, 306–310, 2016.
Koven, C. D., Knox, R. G., Fisher, R. A., Chambers, J. Q., Christoffersen, B. O., Davies, S. J., Detto, M., Dietze, M. C., Faybishenko, B., Holm, J., Huang, M., Kovenock, M., Kueppers, L. M., Lemieux, G., Massoud, E., McDowell, N. G., Muller-Landau, H. C., Needham, J. F., Norby, R. J., Powell, T., Rogers, A., Serbin, S. P., Shuman, J. K., Swann, A. L. S., Varadharajan, C., Walker, A. P., Wright, S. J., and Xu, C.: Benchmarking and parameter sensitivity of physiological and vegetation dynamics using the Functionally Assembled Terrestrial Ecosystem Simulator (FATES) at Barro Colorado Island, Panama, Biogeosciences, 17, 3017–3044, https://doi.org/10.5194/bg-17-3017-2020, 2020.
Lapola, D. M. and Norby, R. J.: Amazon FACE: Assessing the effects of increased atmospheric CO2 on the ecology and resilience of the Amazon forest – Science Plan and Implementation Strategy, Brasilia, Brazil: Ministerio de Ciencia, Technologia e Inovaçao – MCTI, 2014.
Lapola, D. M., Oyama, M. D., and Nobre, C. A.: Exploring the range of climate biome projections for tropical South America: The role of CO2 fertilization and seasonality, Global Biogeochem. Cy., 23, GB30003, https://doi.org/10.1029/2008GB003357, 2009.
Lewis, S. L., Phillips, O. L., Baker, T. R., Lloyd, J., Malhi, Y., Almeida, S., Higuchi, N., Laurance, W. F., Neill, D. A., and Silva, J. N. M.: Concerted changes in tropical forest structure and dynamics: evidence from 50 South American long-term plots, Philos. T. Roy. Soc. B., 359, 421–436, 2004.
Li, Y., Baker, J. C., Brando, P. M., Hoffman, F. M., Lawrence, D. M., Morton, D. C., Swann, A. L., Uribe, M. d. R., and Randerson, J. T.: Future increases in Amazonia water stress from CO2 physiology and deforestation, Nat. Water, 1, 769–777, 2023.
Liu, Y., Parolari, A. J., Kumar, M., Huang, C.-W., Katul, G. G., and Porporato, A.: Increasing atmospheric humidity and CO2 concentration alleviate forest mortality risk, P. Natl. Acad. Sci. USA, 114, 9918–9923, 2017.
Liu, Y. Y., Van Dijk, A. I., De Jeu, R. A., Canadell, J. G., McCabe, M. F., Evans, J. P., and Wang, G.: Recent reversal in loss of global terrestrial biomass, Nat. Clim. Change, 5, 470–474, 2015.
Madani, N., Kimball, J. S., Ballantyne, A. P., Affleck, D. L., Van Bodegom, P. M., Reich, P. B., Kattge, J., Sala, A., Nazeri, M., and Jones, M. O.: Future global productivity will be affected by plant trait response to climate, Sci. Rep., 8, 2870, https://doi.org/10.1038/s41598-018-21172-9, 2018.
Maia, V. A., Santos, A. B. M., de Aguiar-Campos, N., de Souza, C. R., de Oliveira, M. C. F., Coelho, P. A., Morel, J. D., da Costa, L. S., Farrapo, C. L., and Fagundes, N. C. A.: The carbon sink of tropical seasonal forests in southeastern Brazil can be under threat, Sci. Adv., 6, eabd4548, https://doi.org/10.1126/sciadv.abd4548, 2020.
McDowell, N. G., Bond, B. J., Dickman, L. T., Ryan, M. G., and Whitehead, D.: Relationships between tree height and carbon isotope discrimination, in: Size-and Age-Related Changes in Tree Structure and Function, Springer, 255–286, 2011.
McDowell, N., Allen, C. D., Anderson‐Teixeira, K., Brando, P., Brienen, R., Chambers, J., Christoffersen, B., Davies, S., Doughty, C., and Duque, A.: Drivers and mechanisms of tree mortality in moist tropical forests, New Phytol., 219, 851–869, 2018.
McDowell, N. G., Allen, C. D., Anderson-Teixeira, K., Aukema, B. H., Bond-Lamberty, B., Chini, L., Clark, J. S., Dietze, M., Grossiord, C., and Hanbury-Brown, A.: Pervasive shifts in forest dynamics in a changing world, Science, 368, eaaz9463, https://doi.org/10.1126/science.aaz9463, 2020.
McDowell, N. G., Sapes, G., Pivovaroff, A., Adams, H. D., Allen, C. D., Anderegg, W. R., Arend, M., Breshears, D. D., Brodribb, T., and Choat, B.: Mechanisms of woody-plant mortality under rising drought, CO2 and vapour pressure deficit, Nat. Rev. Earth Env., 3, 294–308, 2022.
Needham, J. F., Chambers, J., Fisher, R., Knox, R., and Koven, C. D.: Forest responses to simulated elevated CO2 under alternate hypotheses of size-and age-dependent mortality, Glob. Change Biol., 26, 5734–5753, 2020.
Nölte, A., Yousefpour, R., Cifuentes-Jara, M., and Hanewinkel, M. Sharp decline in future productivity of tropical reforestation above 29° C mean annual temperature, Sci. Adv., 9, eadg9175, https://doi.org/10.1126/sciadv.adg9175, 2023.
Pan, Y., Birdsey, R. A., Fang, J., Houghton, R., Kauppi, P. E., Kurz, W. A., Phillips, O. L., Shvidenko, A., Lewis, S. L., and Canadell, J. G.: A large and persistent carbon sink in the world's forests, Science, 333, 988–993, 2011.
Pan, Y., Jackson, R. B., Hollinger, D. Y., Phillips, O. L., Nowak, R. S., Norby, R. J., Oren, R., Reich, P. B., Lüscher, A., and Mueller, K. E.: Contrasting responses of woody and grassland ecosystems to increased CO2 as water supply varies, Nat. Ecol. Evol., 6, 315–323, 2022.
Papastefanou, P., Zang, C. S., Angelov, Z., de Castro, A. A., Jimenez, J. C., De Rezende, L. F. C., Ruscica, R. C., Sakschewski, B., Sörensson, A. A., Thonicke, K., Vera, C., Viovy, N., Von Randow, C., and Rammig, A.: Recent extreme drought events in the Amazon rainforest: assessment of different precipitation and evapotranspiration datasets and drought indicators, Biogeosciences, 19, 3843–3861, https://doi.org/10.5194/bg-19-3843-2022, 2022.
Peñuelas, J., Ciais, P., Canadell, J. G., Janssens, I. A., Fernández-Martínez, M., Carnicer, J., Obersteiner, M., Piao, S., Vautard, R., and Sardans, J.: Shifting from a fertilization-dominated to a warming-dominated period, Nat. Ecol. Evol., 1, 1438–1445, 2017.
Phillips, O. L., Aragão, L. E., Lewis, S. L., Fisher, J. B., Lloyd, J., López-González, G., Malhi, Y., Monteagudo, A., Peacock, J., and Quesada, C. A.: Drought sensitivity of the Amazon rainforest, Science, 323, 1344–1347, 2009.
Seiler, C., Melton, J. R., Arora, V. K., Sitch, S., Friedlingstein, P., Anthoni, P., Goll, D., Jain, A. K., Joetzjer, E., and Lienert, S.: Are terrestrial biosphere models fit for simulating the global land carbon sink?, J. Adv. Model Earth Sy., 14, e2021MS002946, https://doi.org/10.1029/2021MS002946, 2022.
Sitch, S., Smith, B., Prentice, I. C., Arneth, A., Bondeau, A., Cramer, W., Kaplan, J. O., Levis, S., Lucht, W., and Sykes, M. T.: Evaluation of ecosystem dynamics, plant geography and terrestrial carbon cycling in the LPJ dynamic global vegetation model, Glob. Change Biol., 9, 161–185, 2003.
Skinner, C. B., Poulsen, C. J., and Mankin, J. S. Amplification of heat extremes by plant CO2 physiological forcing, Nat. Commun., 9, 1094, https://doi.org/10.1038/s41467-018-03472-w, 2018.
Smith, W. K., Fox, A. M., MacBean, N., Moore, D. J., and Parazoo, N. C.: Constraining estimates of terrestrial carbon uptake: New opportunities using long-term satellite observations and data assimilation, New Phytol., 225, 105–112, 2020.
Stephenson, N. L. and van Mantgem, P. J. Forest turnover rates follow global and regional patterns of productivity, Ecol. Lett., 8, 524–531, 2005.
Sullivan, M. J., Lewis, S. L., Affum-Baffoe, K., Castilho, C., Costa, F., Sanchez, A. C., Ewango, C. E., Hubau, W., Marimon, B., and Monteagudo-Mendoza, A.: Long-term thermal sensitivity of Earth's tropical forests, Science, 368, 869–874, 2020.
Tao, S., Chave, J., Frison, P.-L., Le Toan, T., Ciais, P., Fang, J., Wigneron, J.-P., Santoro, M., Yang, H., and Li, X.: Increasing and widespread vulnerability of intact tropical rainforests to repeated droughts, P. Natl. Acad. Sci. USA, 119, e2116626119, https://doi.org/10.1073/pnas.2116626119, 2022.
Trugman, A. T., Anderegg, L. D., Wolfe, B. T., Birami, B., Ruehr, N. K., Detto, M., Bartlett, M. K., and Anderegg, W. R.: Climate and plant trait strategies determine tree carbon allocation to leaves and mediate future forest productivity, Glob. Change Biol., 25, 3395–3405, 2019.
Van Der Sleen, P., Groenendijk, P., Vlam, M., Anten, N. P., Boom, A., Bongers, F., Pons, T. L., Terburg, G., and Zuidema, P. A.: No growth stimulation of tropical trees by 150 years of CO−2 fertilization but water-use efficiency increased, Nat. Geosci., 8, 24–28, 2015.
Walker, A. P., De Kauwe, M. G., Bastos, A., Belmecheri, S., Georgiou, K., Keeling, R. F., McMahon, S. M., Medlyn, B. E., Moore, D. J., and Norby, R. J.: Integrating the evidence for a terrestrial carbon sink caused by increasing atmospheric CO2, New Phytol., 229, 2413–2445, 2021.
Wieder, W. R., Cleveland, C. C., Smith, W. K., and Todd-Brown, K.: Future productivity and carbon storage limited by terrestrial nutrient availability, Nat. Geosci., 8, 441–444, 2015.
Winkler, A. J., Myneni, R. B., Hannart, A., Sitch, S., Haverd, V., Lombardozzi, D., Arora, V. K., Pongratz, J., Nabel, J. E. M. S., Goll, D. S., Kato, E., Tian, H., Arneth, A., Friedlingstein, P., Jain, A. K., Zaehle, S., and Brovkin, V.: Slowdown of the greening trend in natural vegetation with further rise in atmospheric CO2, Biogeosciences, 18, 4985–5010, https://doi.org/10.5194/bg-18-4985-2021, 2021.
Wright, S. J., Kitajima, K., Kraft, N. J., Reich, P. B., Wright, I. J., Bunker, D. E., Condit, R., Dalling, J. W., Davies, S. J., and Díaz, S.: Functional traits and the growth–mortality trade-off in tropical trees, Ecology, 91, 3664–3674, 2010.
Yang, H., Ciais, P., Frappart, F., Li, X., Brandt, M., Fensholt, R., Fan, L., Saatchi, S., Besnard, S., and Deng, Z.: Global increase in biomass carbon stock dominated by growth of northern young forests over past decade, Nat. Geosci., 16, 886–892, 2023.
Yao, Y.: ORCHIDEE-CAN-NHA model (r7236), IPSL Data Catalog [code], https://doi.org/10.14768/8C2D06FB-0020-4BC5-A831-C876F5FBBFE9, 2021.
Yao, Y., Joetzjer, E., Ciais, P., Viovy, N., Cresto Aleina, F., Chave, J., Sack, L., Bartlett, M., Meir, P., Fisher, R., and Luyssaert, S.: Forest fluxes and mortality response to drought: model description (ORCHIDEE-CAN-NHA r7236) and evaluation at the Caxiuanã drought experiment, Geosci. Model Dev., 15, 7809–7833, https://doi.org/10.5194/gmd-15-7809-2022, 2022.
Yao, Y., Ciais, P., Viovy, N., Joetzjer, E., and Chave, J.: How drought events during the last century have impacted biomass carbon in Amazonian rainforests, Glob. Change Biol., 29, 747–762, 2023.
Yu, K., Ciais, P., Seneviratne, S. I., Liu, Z., Chen, H. Y., Barichivich, J., Allen, C. D., Yang, H., Huang, Y., and Ballantyne, A. P.: Field-based tree mortality constraint reduces estimates of model-projected forest carbon sinks, Nat. Commun., 13, 2094, https://doi.org/10.1038/s41467-022-29619-4, 2022.
Zhang, K., de Almeida Castanho, A. D., Galbraith, D. R., Moghim, S., Levine, N. M., Bras, R. L., Coe, M. T., Costa, M. H., Malhi, Y., and Longo, M.: The fate of Amazonian ecosystems over the coming century arising from changes in climate, atmospheric CO2, and land use, Glob. Change Biol., 21, 2569–2587, 2015.
Zhu, Z., Piao, S., Myneni, R. B., Huang, M., Zeng, Z., Canadell, J. G., Ciais, P., Sitch, S., Friedlingstein, P., and Arneth, A.: Greening of the Earth and its drivers, Nat. Clim. Change, 6, 791–795, 2016.
Zuidema, P. A., Heinrich, I., Rahman, M., Vlam, M., Zwartsenberg, S. A., and van der Sleen, P.: Recent CO2 rise has modified the sensitivity of tropical tree growth to rainfall and temperature, Glob. Change Biol., 26, 4028–4041, 2020.