the Creative Commons Attribution 4.0 License.
the Creative Commons Attribution 4.0 License.
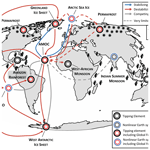
Climate tipping point interactions and cascades: a review
Nico Wunderling
Anna S. von der Heydt
Yevgeny Aksenov
Stephen Barker
Robbin Bastiaansen
Victor Brovkin
Maura Brunetti
Victor Couplet
Thomas Kleinen
Caroline H. Lear
Johannes Lohmann
Rosa Maria Roman-Cuesta
Sacha Sinet
Didier Swingedouw
Ricarda Winkelmann
Pallavi Anand
Jonathan Barichivich
Sebastian Bathiany
Mara Baudena
John T. Bruun
Cristiano M. Chiessi
Helen K. Coxall
David Docquier
Jonathan F. Donges
Swinda K. J. Falkena
Ann Kristin Klose
David Obura
Juan Rocha
Stefanie Rynders
Norman Julius Steinert
Matteo Willeit
Climate tipping elements are large-scale subsystems of the Earth that may transgress critical thresholds (tipping points) under ongoing global warming, with substantial impacts on the biosphere and human societies. Frequently studied examples of such tipping elements include the Greenland Ice Sheet, the Atlantic Meridional Overturning Circulation (AMOC), permafrost, monsoon systems, and the Amazon rainforest. While recent scientific efforts have improved our knowledge about individual tipping elements, the interactions between them are less well understood. Also, the potential of individual tipping events to induce additional tipping elsewhere or stabilize other tipping elements is largely unknown. Here, we map out the current state of the literature on the interactions between climate tipping elements and review the influences between them. To do so, we gathered evidence from model simulations, observations, and conceptual understanding, as well as examples of paleoclimate reconstructions where multi-component or spatially propagating transitions were potentially at play. While uncertainties are large, we find indications that many of the interactions between tipping elements are destabilizing. Therefore, we conclude that tipping elements should not only be studied in isolation, but also more emphasis has to be put on potential interactions. This means that tipping cascades cannot be ruled out on centennial to millennial timescales at global warming levels between 1.5 and 2.0 ∘C or on shorter timescales if global warming surpassed 2.0 ∘C. At these higher levels of global warming, tipping cascades may then include fast tipping elements such as the AMOC or the Amazon rainforest. To address crucial knowledge gaps in tipping element interactions, we propose four strategies combining observation-based approaches, Earth system modeling expertise, computational advances, and expert knowledge.
- Article
(3453 KB) - Full-text XML
-
Supplement
(1253 KB) - BibTeX
- EndNote
- Included in Encyclopedia of Geosciences
1.1 Climate tipping elements
Climate change can cause abrupt and irreversible environmental and societal change (Masson-Delmotte et al., 2021). Several climate subsystems have been identified at risk of undergoing qualitative and often irreversible change when critical thresholds of global warming are transgressed (Wang et al., 2023; Armstrong McKay et al., 2022; Bathiany et al., 2016; Lenton et al., 2008). Such subsystems are termed tipping elements (TEs), and examples include the Atlantic Meridional Overturning Circulation (AMOC), polar ice sheets, tropical rainforests, permafrost regions, and the marine biosphere. Nonlinear changes can occur at tipping points (TPs), where a slight change in a parameter or a small perturbation of a system's state can cause a large change in the system, driving it to transition into a completely different (often undesirable) state. From a dynamical system point of view, a tipping point can be reached when passing a critical value of a control parameter, for example the atmospheric CO2 concentration, which affects the equilibrium states of the system.
In the context of this paper, we refer to a tipping element as any climate subsystem that shows threshold behavior (at its so-called tipping point) beyond which self-amplifying feedbacks to forcing reorganize the system qualitatively (e.g., from an ice-covered to an ice-free state in Greenland). This means that, at the tipping point, a small shift in the background climate can trigger a large-scale qualitative system change. Once the tipping element is triggered, its self-amplifying feedbacks dominate the dynamics of the tipping element during the tipping process. This definition is taken from Levermann et al. (2012) and includes large-scale climate tipping elements such as the AMOC (Weijer et al., 2019) and polar ice sheets (Rosier et al., 2021), where the associated feedbacks (e.g., salt–advection, melt–elevation, or ice–albedo) are well known. Our definition also includes more regional bistabilities between savanna and forest vegetation in the Amazon region. In addition, we also consider elements that can show nonlinear behavior but it is speculated whether they should be considered tipping elements (Armstrong McKay et al., 2022) (El Niño–Southern Oscillation: ENSO, Arctic sea ice, and Indian summer monsoon in this paper). These entities are important due to their connections to tipping elements and for Earth system stability but also due to their potentially dramatic consequences on regional and local scales including impacts on the biosphere and human societies. We therefore do not restrict ourselves to the most plausible tipping elements in this review, but also include nonlinear components like Arctic sea ice, ENSO, and monsoon systems that can act as mediators of tipping events in the Earth system.
Tipping processes involving several tipping elements can also be found in Earth's history: during the last ice age, repeated abrupt shifts, so-called Dansgaard–Oeschger events, occurred between cold and warm phases lasting 1000–4000 years (Dansgaard et al., 1993). While mostly polar and Northern Hemisphere elements (sea ice, ocean circulation, atmospheric dynamics) appear to have been involved (Vettoretti and Peltier, 2016; Zhang et al., 2014; Clement and Peterson, 2008; Ganopolski and Rahmstorf, 2001), the climate impact of these shifts was global (Barbante et al., 2006; Shackleton et al., 2000; Stocker and Johnsen, 2003).
1.2 Interactions in the Earth's climate system
Most climate subsystems are linked via circulation systems in the ocean and atmosphere, which leads to statistical associations between them in their natural variability, often called teleconnections. For example, ENSO, the monsoon systems, and Atlantic multi-decadal variability form global modes of climate variability (Kravtsov et al., 2018; Dommenget and Latif, 2008). In addition, sea surface temperature variability in the North Pacific coupled to tropical variability (ENSO) is transferred to other regions via atmospheric teleconnections and amplified on longer timescales by the large ocean heat capacity (Dommenget and Latif, 2008). Similarly, multi-decadal variability originating in the North Atlantic Ocean (Knight et al., 2005; Delworth and Mann, 2000), which is believed to be partly connected to the AMOC (Buckley and Marshall, 2016), has a global expression in sea surface temperature patterns due to the interaction of slow oceanic and fast (but large-scale) atmospheric processes (Kravtsov et al., 2018). Hence most tipping elements and other nonlinear components (such as ENSO or Arctic sea ice) are not isolated form each other but are either connected directly or mediated via changes to the background state (Liu et al., 2023; Kriegler et al., 2009). Via such connections between tipping elements (see Fig. 1), tipping in one subsystem – the leading subsystem – can therefore cause tipping in another one – the following subsystem (Klose et al., 2020; Dekker et al., 2018). Here we call the linkages between tipping elements and/or other nonlinear components tipping interactions, whether they have a stabilizing or a destabilizing effect. The most extreme case is the situation in which the tipping of element A causes a subsequent tipping of element B. In this paper, we define a sequence of events involving several nonlinear components of the Earth system as tipping cascades (Dekker et al., 2018; Wunderling et al., 2021a). These tipping cascades can come in various forms dependent on the ordering of tipping elements (e.g., Klose et al., 2021) and can be different depending on the bifurcations (e.g., Hopf or fold bifurcation) present in the individual tipping elements (Dekker et al., 2018). Eventually a tipping cascade might result in a fundamental change in the Earth’s equilibrium climate. It is important to note that interactions between tipping elements are not all monotonically constant but may also change depending on the current state of the involved tipping elements (e.g., interactions can be nonstationary) or may affect different parts of a particular tipping element in a different way. Since knowledge at this level of detail in the interactions is very heterogeneous and sparse, we will use the above definition of tipping linkages and cascades in this paper.
For example, a disintegration of the Greenland Ice Sheet can lead to an abrupt AMOC shift, while an abrupt change in AMOC strength can lead to an intensification of ENSO. We do not restrict our definition to specific spatial scales, timescales, or severity of impact of the tipping elements. Therefore, the slow local invasion fronts in spatial (eco)systems would also be considered, leading to a rapid local change in response to the slow system-wide and large-scale changes (e.g., Bel et al., 2012). Interactions between climate tipping elements could effectively lower the thresholds for triggering a tipping event or cascade compared to individual tipping elements (Wunderling et al., 2021a; Klose et al., 2020). Moreover, a tipping cascade could activate processes leading to additional CO2 emissions into the atmosphere; permafrost thaw and forest dieback are typical examples of such feedbacks (Wunderling et al., 2020; Lenton et al., 2019; Steffen et al., 2018).
Due to the many nonlinearities in the climate system, it is also conceivable that components of the Earth system, though not necessarily tipping elements on their own, could mediate or amplify nonlinear transitions in one component, also creating larger-scale impacts in other components. A prominent example is Arctic summer sea ice cover, which shows an almost linear response to the CO2 forcing and is not expected to show tipping behavior under anthropogenic forcing (Lee et al., 2021; Notz and Stroeve, 2016). Nevertheless it can still sharpen and amplify transitions in the ocean–atmosphere–cryosphere system (Gildor and Tziperman, 2003). Note, however, that Arctic winter sea ice extent does show very different states at the same atmospheric CO2 concentration (Schwinger et al., 2022) and may be considered a tipping element (Hankel and Tziperman, 2023). Because Arctic summer and winter sea ice interacts with the AMOC, we take both of them into account as one node in our network.
On the other hand, an abrupt transition in one tipping element may also stabilize other climate subsystems (e.g., Nian et al., 2023; Sinet et al., 2023) as is the case for a weakening AMOC decreasing local temperatures around Greenland (Jackson et al., 2015).
1.3 Motivation and structure of this work
While most TEs that have been proposed so far are clearly regional (with some being large-scale), there are significant knowledge gaps with respect to their tipping probability, impact estimates, timescales, and interactions. The main reasons for these knowledge gaps and therefore large uncertainties, are among others, (i) that experiments from comprehensive and process-based models are sparse (however, future projects plan to systematically increase the number of such experiments such as TIPMIP1 and WhatifMIP). (ii) Since recent observations of large-scale tipping processes (e.g., in Greenland and Antarctic ice sheets or the Amazon rainforest) are not available, we need to rely on (iii) paleoclimatic data, which are inherently sparse in availability. Due to these uncertainties, the potential of a tipping cascade that could lead to a global reorganization of the climate system (Steffen et al., 2018; Hughes et al., 2013) remains speculative. However, since multiple individual tipping point thresholds may be crossed during this century with ongoing global warming and lead to severe tipping element interactions and cascading transitions in the worst case, it is critical to review the current state of the science and reveal research gaps that need to be filled in Armstrong McKay et al. (2022), Masson-Delmotte et al. (2021), and Rocha et al. (2018). Therefore, we provide an overview here of the current knowledge of tipping element interactions and the potential for tipping cascades. We review individual interactions between the tipping elements and determine whether interactions tend to stabilize or destabilize the climate system. Furthermore, we explain why tipping cascades are plausible using past observed cascades and a recent example. Finally, we discuss the state of the literature on tipping cascade likelihoods and how scientific work can improve risks assessments for cascading transitions.
The main part of this paper reviews the current knowledge of interactions between specific pairs of tipping components (Sect. 2). In Sect. 3, we discuss three paleoclimate candidates of tipping sequences that involve more than one tipping element: one from the more distant past (Eocene–Oligocene transition; approximately 34 million years ago), one from the more recent past (Dansgaard–Oeschger events, Bølling–Allerød warm period, and Heinrich events; during and since the last glacial period), and a paleoclimatic perspective on interactions between the AMOC and Amazon rainforest. Next, we map out the present state of modeling tipping sequences with respect to the role of complex Earth system models and more conceptualized approaches (Sect. 4). Lastly, we discuss current research gaps and ways forward from a knowledge, modeling, and data perspective. We also discuss the value of newly arising methods from machine learning and Earth observation and how they could complement the present research on interacting climate tipping elements. Finally, we conclude with recent progress on tipping cascades and interactions between tipping elements (both Sect. 5). Each of the sections (Sects. 1–5 and Sects. 2.1–2.7, 3.1–3.3) in this paper have been written by a different group of experts in the respective field (the co-authors of this study).
2.1 Interactions across scales in space and time
In this section, we lay out the current state of the literature on the interaction processes between components that are known to show nonlinear behavior or are even suspected tipping elements. The summary of Sect. 2.2–2.8 is shown in Table 1 and Figs. 1, 2, and 3. We show that these elements are not isolated entities but interact across the entire globe (Fig. 1). Not only do the interactions span global distances, but the elements themselves are also systems of the sub-continental up to (nearly) global spatial scale that may tip on temporal scales of months up to millennia; i.e., tipping elements interact across scales in space and time (Fig. 2) (Rocha et al., 2018; Kriegler et al., 2009). The respective processes of the interactions can be found in Table 1 (summarized in Fig. 3), alongside an estimation of the interaction direction and, if available, an estimation of their strength (based on the detailed literature review of Sect. 2.2–2.8).
Some tipping elements are of sub-continental spatial scale (e.g., coral reefs or the Greenland Ice Sheet), while others cover significant portions of the globe (e.g., AMOC). Also, the temporal scales differ vastly among the different climate tipping elements: some of them are considered fast tipping elements once a tipping process has been initiated (tipping on the order of months, to years or decades, to centuries, e.g., Amazon rainforest and AMOC), while others are considered slow tipping elements (tipping on the order of centuries to millennia, e.g., Greenland Ice Sheet). These individual dynamics in space and time of the individual tipping elements are therefore also important for their interactions (mapped out in Fig. 2).
Weijer et al. (2019)Mecking et al. (2016)Stouffer et al. (2007)Swingedouw et al. (2009)Swingedouw et al. (2008)Stouffer et al. (2007)Seidov et al. (2005)Jackson et al. (2015)Stouffer et al. (2006)Bintanja et al. (2013)Swingedouw et al. (2008)Gomez et al. (2020)Kopp et al. (2010)Gomez et al. (2020)Mitrovica et al. (2009)Liu and Fedorov (2022)Li et al. (2021)Jansen et al. (2020)Sévellec et al. (2017)Liu and Fedorov (2022)Liu et al. (2020)Delworth et al. (2016)Wunderling et al. (2020)Hošeková et al. (2021)Casas-Prat and Wang (2020)Pedersen and Christensen (2019)Ben-Yami et al. (2024)Bellomo et al. (2023)Jackson et al. (2015)Parsons et al. (2014)Orihuela-Pinto et al. (2022b)Dekker et al. (2018)Timmermann et al. (2007)Kim et al. (2023)Ayarzagüena et al. (2018)Duque-Villegas et al. (2019)Jiménez-Muñoz et al. (2016)Scott et al. (2019)Paolo et al. (2018)Nicolas et al. (2017)Muñiz-Castillo et al. (2019)Lough et al. (2018)Veron et al. (2009)Orihuela-Pinto et al. (2022a)Wassenburg et al. (2021)Swingedouw et al. (2009)de Vrese et al. (2023)Nitzbon et al. (2020)Table 1List of links between elements (tipping elements and other nonlinear components) discussed in this paper. We list directed links with a short summary of the main physical mechanism(s) underlying the specific connection. We estimate whether the effect is stabilizing or destabilizing the impacted element. Because uncertainties in tipping linkages are large, we assess the uncertainty and the level of confidence following IPCC guidelines using the agreement among different studies as well as the overall evidence (Mastrandrea et al., 2011). If there is incipient evidence in the literature, we also add an indication of the qualitative strength of the response (weak, moderate, strong). Otherwise the link and/or its strength is set as unclear. Lastly, key references for the specific link are noted. Abbreviations: AABW – Antarctic Bottom Water, AMAZ – Amazon rainforest, AMOC – Atlantic Meridional Overturning Circulation, ENSO – El Niño–Southern Oscillation, GIS – Greenland Ice Sheet, ISM – Indian summer monsoon, PERM – permafrost, SST – sea surface temperature, WAIS – West Antarctic Ice Sheet, WAM – West African monsoon.
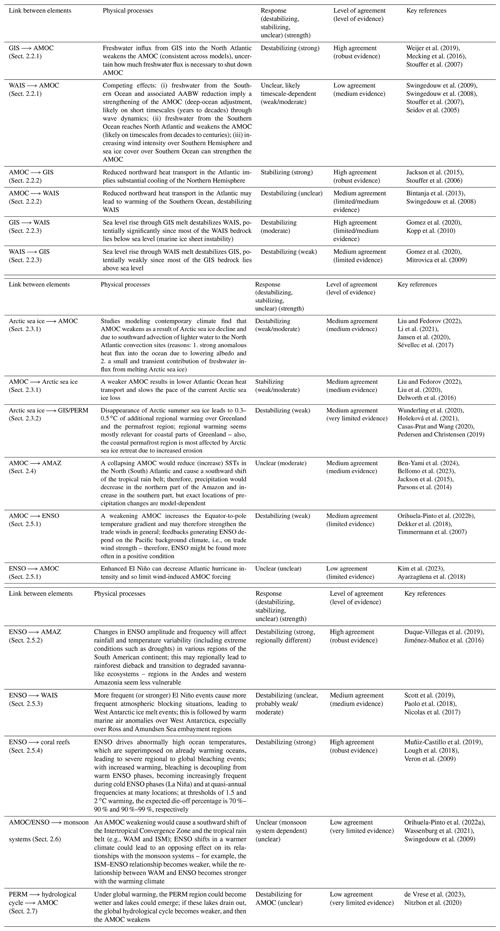
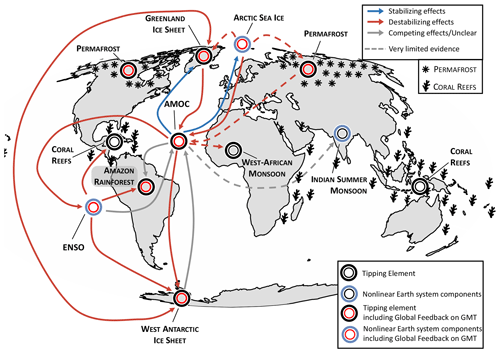
Figure 1Interactions between tipping elements on a world map. All tipping elements discussed in this review article are shown together with their potential connections. The causal interactions links can have stabilizing (blue), destabilizing (red), or unclear (gray) effects. For some elements, it is speculative whether they are tipping elements on their own (such as ENSO or Arctic sea ice) and they are denoted as such (blue outer ring) but they are included as nonlinear Earth system components if they play an important role in mediating transitions towards (or from) core tipping elements (compare Sect. 1.2). Tipping elements that exert a notable feedback on global mean temperature when they tip are denoted by a red inner ring. This temperature feedback can be positive (i.e., amplifying warming, as likely for the permafrost, the Arctic sea ice, the Greenland and West Antarctic ice sheets, the Amazon rainforest, and ENSO) or negative (i.e., dampening warming, as likely for the AMOC).
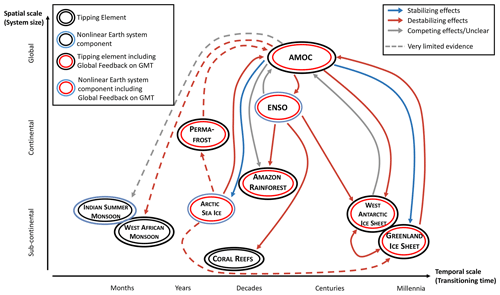
Figure 2Interactions between tipping elements across scales in space and time. Temporal scales are transitioning times of a disintegrating tipping element from months up to millennia. Spatial scales denote the system size from sub-continental to (nearly) global scales. Transitioning times are taken from Armstrong McKay et al. (2022), and spatial scales from Winkelmann et al. (2022). The causal links can be stabilizing (blue), destabilizing (red), or unclear (gray). Some tipping elements are particularly speculative (such as ENSO or the Arctic sea ice) and denoted as nonlinear Earth System components (blue border). Tipping elements that exert a feedback on the global mean temperature when they tip are depicted with an additional red inner circle.
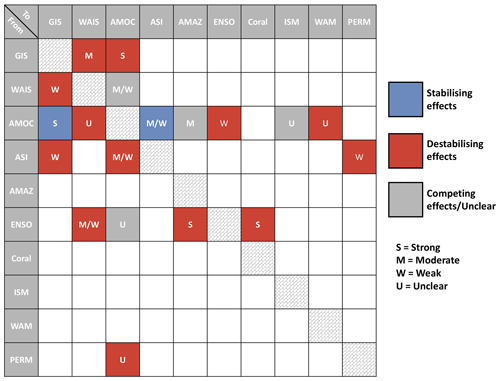
Figure 3Interactions between tipping elements and other nonlinear components. Summary matrix of interactions in Figs. 1 and 2. More details on the specific interactions can be found in Table 1 and Sect. 2.2–2.7. Columns denote the element from which the interaction originates, and rows denote the tipping element which is affected by the interaction. We separate three different types of effects: a stabilizing link (blue box), a destabilizing link (red box), and an unclear or competing link (gray box). White boxes denote no (or an unknown) link. The strengths of the links are separated into four groups: strong (S), moderate (M), weak (W), and unclear (U) if a strength estimate is lacking.
2.2 Interactions between ice sheets and AMOC
The AMOC, Greenland Ice Sheet (GIS), and West Antarctic Ice Sheet (WAIS) are core tipping elements and are threatened by increasing CO2 emissions (Armstrong McKay et al., 2022; IPCC, 2019). Moreover, GIS, AMOC, and WAIS interact on very different timescales ranging from decades to multiple centuries. While some of those links might be stabilizing, others are destabilizing and would allow for the possibility of large-scale cascading events.
2.2.1 Effects of disintegrating ice sheets on AMOC
Greenland Ice Sheet to AMOC. The AMOC depends on the formation of dense water in the high latitudes of the North Atlantic. In its present state, this process is widely sustained by the positive salt–advection feedback (Weijer et al., 2019; Rahmstorf, 1996; Stommel, 1961) – as the AMOC transports salt northward, a higher surface water density is maintained in this region. As GIS melting increases, the associated discharge of freshwater in the ocean would result in a decrease in the surface water density, inhibiting the formation of dense waters through deep convection and thereby weakening the circulation. As less salt is transported to the North Atlantic, the salt–advection feedback implies a self-sustained freshening of the high latitudes of the North Atlantic, which, in the worst case, can result in the collapse of the AMOC. On top of this classical positive feedback, there is a wide range of other feedbacks related to the AMOC, either negative (heat advection feedback, e.g., Swingedouw et al., 2007) or positive (evaporation feedback). An overall destabilizing impact of GIS melting on the AMOC is mostly consistent across models, where adding freshwater in the North Atlantic (e.g., Jackson and Wood, 2018; Mecking et al., 2016; Stouffer et al., 2007), also in combination with increasing CO2 emissions (Bakker et al., 2016; Hu et al., 2013; Swingedouw et al., 2006), leads to a substantial weakening of the circulation. Importantly, in the case of a collapse of the AMOC, some models suggest that the AMOC does not recover within a human timescale (Jackson and Wood, 2018; Mecking et al., 2016). At the moment, one of the key limitations relating GIS melting and the respective AMOC response concerns the way meltwater is spread along Greenland towards the open ocean. This lateral diffusion is mainly performed by oceanic eddies, whose spatial scale is of the order of 10 km at those latitudes, and necessitates oceanic resolution in AOGCMs (atmosphere–ocean general circulation models) of the order of 2–3 km to be properly resolved. As a consequence, the spread of GIS meltwater towards the convection sites in the Labrador, Irminger, and Nordic seas as well as the Atlantic Subpolar Gyre might be underestimated in AOGCMs, which might strongly diminish the potential impact of GIS melting on the AMOC (Martin and Biastoch, 2023; Liu and Fedorov, 2022; Swingedouw et al., 2022; Born and Stocker, 2014). Thus, while there have been a few attempts to couple AOGCMs with ice sheet models (e.g., Madsen et al., 2022; Kreuzer et al., 2021; Ackermann et al., 2020; Muntjewerf et al., 2020), the simulated impact of GIS melting on the AMOC remains moderate but might be underestimated.
West Antarctic Ice Sheet to AMOC. In the case of a freshwater release in the Southern Hemisphere originating from West Antarctica, different opposing processes are at play that could affect the AMOC. These effects have been identified to act on different timescales and depend on the state of the circulation (Berk et al., 2021; Swingedouw et al., 2009). First, the weakening of AABW formation might lead to enhancement of the AMOC through the so-called ocean bipolar seesaw related to deep-ocean adjustment through oceanic large-scale waves (Stocker and Johnsen, 2003; Pedro et al., 2018). Second, the increase in wind intensity over the Southern Hemisphere, related to an increase in sea ice cover (Li et al., 2023; Swingedouw et al., 2008), might also help to enhance the AMOC. Third, if large enough, the release of freshwater in the Southern Ocean might eventually reach the North Atlantic on a longer timescale (centuries), possibly weakening the AMOC. As a result, the impact of a WAIS collapse on the AMOC is still unclear, as most models show either a slight weakening (e.g., Stouffer et al., 2007; Seidov et al., 2005) or a slight strengthening (e.g., Swingedouw et al., 2009) of the circulation. Notably, some studies also found that a sufficient freshwater release into the Southern Ocean allows for delaying an AMOC collapse (Sadai et al., 2020), recovering from it (Weaver et al., 2003), or even avoiding it (Sinet et al., 2023). In most cases, the impact of the WAIS melting on the AMOC remains moderate and mainly affects the southern part of the AMOC.
2.2.2 Effects of a collapsing AMOC on ice sheets
An AMOC collapse would imply decreased northward heat transport, leading to a substantial cooling of the Northern Hemisphere, along with warming in the Southern Hemisphere (Pedro et al., 2018; Jackson et al., 2015; Stouffer et al., 2006; Stocker and Johnsen, 2003). Cooling the high latitudes of the North Atlantic would stabilize the GIS, possibly allowing for a safe overshoot of the GIS tipping point (Wunderling et al., 2023; Ritchie et al., 2021). Conversely, the related warming of the Southern Ocean represents a destabilizing impact on the WAIS, being susceptible to these warmer ocean waters via the ice shelves and their buttressing effect on upstream ice flow (Sutter et al., 2023; Favier et al., 2014; Joughin et al., 2014).
2.2.3 Direct interactions between Greenland and West Antarctic ice sheets via sea level feedbacks
It is known that an increase in sea level has an overall destabilizing influence on marine-based sectors of ice sheets, possibly triggering or enhancing the retreat of their grounding line (Schoof, 2007; Weertman, 1974). In the case of an ice sheet collapse, the induced sea level rise from ice sheets would vary locally depending on gravitational effects, rotational effects, and mantle deformation (Kopp et al., 2010; Mitrovica et al., 2009). Overall, sea level rise is expected to negatively impact both the GIS and WAIS, but more strongly the latter where most of the bedrock lies well below sea level (Gomez et al., 2020).
2.3 Arctic sea ice interactions
2.3.1 Interactions between AMOC and Arctic sea ice
The strength of the AMOC is controlled by the deep convective activity at different sites in the North Atlantic Ocean (Labrador, Irminger, and Nordic seas), which is largely driven by its high surface density (Kuhlbrodt et al., 2007).
Changing Arctic sea ice cover can modulate the latter, and thus the AMOC, mainly in two ways (Sévellec et al., 2017). First, it alters radiative heating and ocean–atmosphere heat loss via changing albedo. More precisely, as the Arctic sea ice area has substantially decreased in the past 40 years, especially during summer months (Masson-Delmotte et al., 2021), the open-water fraction of the Arctic Ocean has increased and will continue to do so in the future (Crawford et al., 2021). This has led to an increase in the absorption of solar radiation and to subsequent ocean warming, which can propagate to convection areas. Second, changes in Arctic sea ice alter the ocean density by brine rejection during sea ice formation or conversely by freshening from sea ice melt. In particular, the recent decrease in Arctic sea ice area, together with the ice loss from the Greenland Ice Sheet (Sect. 2.2.1), has added freshwater to the Arctic Ocean, although the trend in freshwater content has slowed down during the past decade (Solomon et al., 2021). According to model simulations performed by Sévellec et al. (2017), these warm and fresh anomalies coming from sea ice melting could propagate southward to the subpolar North Atlantic Ocean. These would affect the deep ocean at the main convection sites by reducing the surface density and would thus weaken the AMOC. The estimated timescale of this propagation is multi-decadal (Liu and Fedorov, 2022; Li et al., 2021; Sévellec et al., 2017).
The AMOC can also affect Arctic sea ice via the transport of warm water to the North Atlantic Ocean and subsequently to the Arctic Ocean via the Barents Sea opening and Fram Strait. A weaker AMOC could result in lower ocean heat transport and increased Arctic sea ice area (Delworth et al., 2016). The estimated timescale of this effect is approximately 1 year (Liu and Fedorov, 2022). However, recent observations show that the ocean heat transport to the Arctic has increased, especially on the Atlantic side (Docquier and Koenigk, 2021; Polyakov et al., 2017; Onarheim et al., 2015; Årthun et al., 2012). Thus, the effect of a decreasing AMOC may merely slow down the pace of ongoing increases in ocean heat transport and the associated decrease in Arctic sea ice (Liu et al., 2020). Despite this tight link between the AMOC and Arctic sea ice, Arctic summer sea ice cannot be considered a tipping element. A tipping point in the Arctic Ocean would mean that it loses so much sea ice that the reduced albedo results in enough warming to prevent sea ice from forming again once melted. However, according to model simulations in which a summer ice-free Arctic Ocean is simulated, Arctic sea ice recovers within 2 years, suggesting that the ice–albedo feedback is alleviated by large-scale recovery mechanisms (Tietsche et al., 2011). Winter sea ice extent does show very different states at the same atmospheric CO2 concentration, attributed to AMOC strength, and may therefore be considered a tipping element (Hankel and Tziperman, 2023; Schwinger et al., 2022).
2.3.2 Effect of Arctic sea ice on the Greenland Ice Sheet and permafrost
Besides an interaction with AMOC, melting Arctic sea ice cover has a direct effect via regional warming on further high-latitude tipping elements such as the Greenland Ice Sheet and the permafrost. In the case of Arctic summer sea ice loss, which may occur during the second half of this century (Niederdrenk and Notz, 2018), additional warming levels caused by this loss are on the order of 0.3–0.5 ∘C regionally over Greenland and the permafrost (Wunderling et al., 2020). Regional warming levels may be higher if Arctic winter sea ice additionally disappears. Further, it has been found that partial Arctic sea ice loss has a limited effect for Greenland warming patterns and is mainly relevant for coastal parts of Greenland (Pedersen and Christensen, 2019).
At the same time, Arctic sea ice loss leads to increased coastal permafrost erosion (Nielsen et al., 2022; Hošeková et al., 2021; Casas-Prat and Wang, 2020; Nielsen et al., 2020), which operates as follows: (1) abrupt changes in summer–autumn sea ice retreat from the permafrost coast lead to (2) an increase in waves, resulting in (3) abrupt increases in erosion rates (2–4 times higher). Thus, (4) there is a potential cascading risk of carbon releases locally to the ocean and atmosphere due to the coastal permafrost collapse (e.g., Nielsen et al., 2022). For more details, see the Supplement (Fig. S1).
2.4 Effects of AMOC changes on the Amazon rainforest
The strength of the AMOC exerts a substantial influence on the climate of tropical South America, most importantly on rainfall and its seasonal distribution. This in turn affects the state and stability of another potential tipping element in the Earth system: the Amazon rainforest.
The most important large-scale effect of the AMOC on rainfall in the Amazon works via the pattern of SSTs in the Atlantic and the associated shifts in the ITCZ (Intertropical Convergence Zone) and the tropical rain belt. There is widespread agreement that a reduction or even collapse of the AMOC would lead to reduced SSTs in the North Atlantic and increased SSTs in the South Atlantic (Bellomo et al., 2023; Manabe and Stouffer, 1995). This change is caused by the reduction in the AMOC-related northward ocean heat transport and is amplified by wind–evaporation feedbacks (Orihuela-Pinto et al., 2022a). The changed SST pattern in turn affects atmospheric circulation by strengthening the Northern Hemisphere Hadley cell, particularly during boreal winter (Bellomo et al., 2023). As the location of the tropical rain belt depends on the cross-equatorial energy flux and the atmosphere energy input close to the Equator (Bischoff and Schneider, 2014; Schneider et al., 2014), a weakened AMOC together with a persistent Southern Ocean warming lead to a southward migration of the tropical rain belt, depending on the CO2 forcing trajectory (Kug et al., 2022). Hence, AMOC weakening may cause a tropical rain belt shift. This southward shift would cause a substantial reduction in rainfall over northern South America and an increase in rainfall over the portion of the Amazon located in the Southern Hemisphere, as well as over northeastern Brazil, which is directly affected by the tropical rain belt (Jackson et al., 2015). Nevertheless, over the Amazon basin, the extent of this migration is model-dependent (e.g., Swingedouw et al., 2013; Stouffer et al., 2006). Indeed, while the northern part of the Amazon might experience a decrease in precipitation, the southern part, in contrast, might see enhanced precipitation, which has the potential to stabilize the rainforest there (Ciemer et al., 2021) with consequences for the carbon inventory (Bozbiyik et al., 2011). The limit between the two regions is where model dependency is strongest, resulting in a large uncertainty concerning the potential impact of AMOC weakening in the Amazon rainforest dieback.
To conclude, although different Earth system models have different biases in the location, shape, and strength of the tropical rain belt, they generally agree on the AMOC-collapse-induced increase in precipitation over the southern portion of the Amazon and northeastern Brazil (Bellomo et al., 2023; Nian et al., 2023; Orihuela-Pinto et al., 2022a; Liu et al., 2020). Given that the forests in the southern half of the basin contribute mostly to the rainfall generation over the basin (Staal et al., 2018), one could speculate that this would lead to a stabilization of the Amazon, given that a substantial fraction (24 %–70 %, Baudena et al., 2021, and references therein) of the rainfall of the basin is nonetheless produced by local moisture recycling. Furthermore, it has been shown that the altered tropical rain belt dynamics throughout the year would mean a reduction of rainfall mostly during the current wet season (peaking around March) and an increase in the dry season, peaking around September (Campos et al., 2019; Parsons et al., 2014). Importantly, the consequences for the rainforest of a more equalized annual cycle are unclear. More generally, the full spectrum of rainforest stressors including societal-driven pressures, such as land use changes driving deforestation, has to be taken into account when assessing AMOC effects over the Amazon rainforest (Lovejoy and Nobre, 2018).
2.5 Influences of ENSO on proposed tipping elements
The El Niño–Southern Oscillation (ENSO) is the most important mode of climate variability on interannual timescales, fundamentally affecting regional and global atmospheric and oceanic circulation (McPhaden et al., 2006). The response to climate change of ENSO itself still remains debated, mainly because there are multiple (positive and negative) feedback processes in the tropical Pacific ocean–atmosphere system, whose relative strength determines the response of ENSO variability (Timmermann et al., 2018; Cai et al., 2015). Further, recent studies disagree about the future frequency of El Niño phases under global warming (Cai et al., 2021; Wengel et al., 2021). In particular, a decreasing frequency of El Niño phases under global warming was suggested by a global climate model resolving mesoscale oceanic eddies and consequently reduced biases in the tropical oceanic mean state (Wengel et al., 2021). Although it is debated or even unlikely whether ENSO should be considered a tipping element itself (Armstrong McKay et al., 2022), it exerts important feedbacks on other global tipping elements. Through its global teleconnections, ENSO has the potential to influence multiple Earth system components including the AMOC, the Amazon rainforest, and the West Antarctic Ice Sheet. Changes in ENSO amplitude or frequency could alter the strength of (stabilizing or destabilizing) feedbacks within other (remote) tipping elements. Therefore, in this section, we discuss possible interactions between ENSO and other tipping elements.
2.5.1 Interactions between ENSO and AMOC
Various physical mechanisms have been discussed to explain how a decline in strength or complete shutdown of the AMOC could affect ENSO variability, mostly in terms of the amplitude of ENSO. An AMOC decline typically leads to cooling in North Atlantic surface temperatures, which affects the global atmospheric circulation, including the trade winds in the tropical Pacific. In many GCMs, upon decline of the AMOC, the northeasterly trade winds are intensified and the Intertropical Convergence Zone (ITCZ) is displaced southwards, eventually leading to an intensification of ENSO amplitude through nonlinear interactions (Timmermann et al., 2007). While the response of the trade winds and ITCZ to AMOC decline seems to be relatively robust within different (generations of) GCMs, the response in ENSO magnitude or frequency is much more model-dependent: trade winds can also affect the thermocline depth in the eastern tropical Pacific, thereby weakening the ENSO (Timmermann et al., 2007). By that, the zonal structure of winds and stratification is affected, leading to zonal shifts of variability patterns but no significant change in amplitude (Williamson et al., 2018). Alternatively, weaker air–sea coupling due to altered trade winds affects the relevant tropical Pacific feedback balance such that the growth rate of ENSO is significantly reduced (Orihuela-Pinto et al., 2022b). In another model study, where physically based, conceptual models of the AMOC (Stommel box model, Stommel, 1961) and ENSO (Zebiak–Cane model, Zebiak and Cane, 1987) are coupled via the trade wind strength, it was found that an AMOC collapse intensifies the tropical Pacific trade winds and shifts the ENSO system further into its oscillatory mode (i.e., amplitude increase) (Dekker et al., 2018). It should be noted that most GCMs still exhibit severe biases in tropical temperature patterns, partly caused by oceanic mesoscale processes that are not properly resolved (Wengel et al., 2021), which complicates the understanding of the fate of ENSO under greenhouse gas increase, but also under AMOC changes.
The reversed pathway, i.e., ENSO impacting the AMOC, likely also exists, but also depends on several atmosphere–ocean processes which may not be adequately resolved in models. A relatively robust teleconnection exists between an El Niño event and the negative phase of the North Atlantic Oscillation (NAO) in (late) winter (Ayarzagüena et al., 2018; Brönnimann et al., 2007). The statistical relationship between the AMOC and the NAO in GCMs depends on the subpolar North Atlantic background state; the AMOC is less sensitive in models that have extensive sea ice cover in deep-water formation areas, while in models with less sea ice cover, the background upper-ocean stratification largely determines how sensitively the AMOC reacts to surface buoyancy forcing (Kim et al., 2023). As for ENSO, the unbiased representation of the North Atlantic mean state represents a significant challenge for GCMs, in part due to insufficient resolution of mesoscale ocean eddies.
2.5.2 Influences of ENSO on the Amazon rainforest
The frequency and amplitude of ENSO variability have changed on decadal to centennial timescales in the past (Cobb et al., 2013). In recent years, extreme El Niño events combined with global warming have become increasingly associated with unprecedented extreme drought and heat stress across the Amazon basin (Jiménez-Muñoz et al., 2016), leading to increases in tree mortality, fire, and dieback (Nobre et al., 2016). Imposing the surface temperature pattern of a typical El Niño event in a global atmosphere–vegetation model suggests increased drought and warming in the Amazon rainforest region (Duque-Villegas et al., 2019), which could enhance rainforest dieback and transition to degraded and fire-prone, savanna-like ecosystems in some regions. This could turn further parts of the Amazon rainforest from a carbon sink to a carbon source.
These destabilizing effects from ENSO on the Amazon rainforest are overlaid with direct climate change effects, land use change, deforestation, and human-made fires in the Amazon rainforest because the Amazon rainforest produces much of its own rainfall (e.g., Staal et al., 2020; Aragão, 2012). Parts of the Amazon rainforest have already turned from a carbon sink to a carbon source (Gatti et al., 2021).
2.5.3 Influences of ENSO on the WAIS
Recent significant surface melt events on West Antarctica were associated with strong El Niño phases (Scott et al., 2019; Nicolas et al., 2017). It has been proposed that these melt events were caused by atmospheric blocking, eventually leading to warm air temperature anomalies over West Antarctica that pass the melt point of parts of the ice sheet (Scott et al., 2019). Using reanalysis data, satellite observations, and hindcasting methods, strong indications have been found that the Ross and Amundsen Sea embayment regions are most affected by El Niño phases (Scott et al., 2019; Deb et al., 2018). In addition, it has been observed that, while ice shelves experience an increase in height (because accumulation height gains exceed basal melt height losses), they suffer from a decrease in mass (because basal ice loss exceeds ice gain from accumulation) due to increased ocean melting during significant El Niño occurrences in the Amundsen and Ross Sea area (Paolo et al., 2018). Further, it is important to note that El Niño phases are not immediately transferred to surface melting in Antarctica but only after some time lag on the order of months (Donat-Magnin et al., 2020).
Taken together, this adds to the growing body of literature indicating that a disintegration of the West Antarctic Ice Sheet, especially along the Ross–Amundsen sector, would be favored by strong El Niño phases and tipping risks may increase if El Niño phases become more frequent or intense under ongoing climate change (Cai et al., 2021; Wang et al., 2017; Cai et al., 2014). This may be concerning in particular because the Amundsen region is where the most vulnerable glaciers of the West Antarctic Ice Sheet are located such as the Pine Island or Thwaites glacier (Favier et al., 2014; Joughin et al., 2014).
2.5.4 Influences of ENSO on warm-water coral reefs
ENSO drives abnormally high sea surface temperatures (seasonal heat waves above summer maxima baselines), which are superimposed on already warming oceans. Anomalous heat destabilizes the relationship between host corals and their symbiotic dinoflagellate algae (zooxanthellae), resulting in severe bleaching and mortality across multiple species of corals on spatial scales exceeding thousands of kilometers. While ENSO is geographically modulated by other ocean dipoles (e.g., Atlantic oscillation, Indian Ocean) (Houk et al., 2020; Krawczyk et al., 2020; Zhang et al., 2017), the Pacific signal is dominant and El Niño warm phases have been related to global episodes of extreme heat stress since the 1970s, e.g., 1979–1980, 1997–1998, and 2014–2017 (Krawczyk et al., 2020; Muñiz-Castillo et al., 2019; Lough et al., 2018; Le Nohaïc et al., 2017). As global warming progresses and oceans become significantly warmer, the incidence of mass bleaching is decoupling from the El Niño warm phase (Veron et al., 2009), with warmer conditions compared to 3 decades ago (McGowan and Theobald, 2023; Muñiz-Castillo et al., 2019). The global recurrence of bleaching has been reduced to an average of 6 years (Hughes et al., 2018), sooner than expected from climate models and satellite-based sea temperatures. With warming temperatures and shortened intervals between major bleaching, multiple human stressors, and ocean acidification, the recovery time for mature assemblages of corals is now insufficient across most regions (Hughes et al., 2018). At the scale of the Great Barrier Reef the emission of volatile sulfur compounds by corals adds to the local atmospheric aerosol load, increasing low-level cloud albedo and reducing warming (Jackson et al., 2018). This breaks down during physiological stress and bleaching, potentially reinforcing thermal stress in a positive feedback loop. The potential contribution of this biologically derived feedback loop to local clouds, sea surface temperature, and coral bleaching is uncertain; this, however, needs validation in other locations and determination of any contribution to climatic conditions at larger spatial and temporal scales. While recovery from repeated bleaching events has been observed (Palacio-Castro et al., 2023; Obura et al., 2018), the thresholds of global mean warming of 1.5 ∘C (70 %–90 % loss of coral reefs globally) and 2 ∘C (90 %–99 % loss) appear to still hold (Lough et al., 2018; Schleussner et al., 2016; Frieler et al., 2013).
2.6 Effects of AMOC and ENSO changes on tropical monsoon systems
Future climate projections show a weakening of the AMOC, which can be substantial in its impact on the regional and global climate via ocean–atmosphere connection (IPCC, 2019). Evidence from modeling and paleo-reconstructions has shown interhemispheric, low- to high-latitude climate connections via ocean–atmosphere linkage for heat and moisture transport (e.g., Nilsson-Kerr et al., 2022; Orihuela-Pinto et al., 2022a; Clemens et al., 2021; Shin and Kang, 2021).
As described above, AMOC weakening leads to a southward shift of the ITCZ (Defrance et al., 2017; Swingedouw et al., 2013; Stouffer et al., 2006), which impacts the various monsoon systems worldwide (Chemison et al., 2022), as also visible in paleorecords (e.g., Sun et al., 2012). For instance, the West African monsoon would strongly decrease for the West African part due to AMOC collapse (Defrance et al., 2017). Further, Nilsson-Kerr et al. (2019) compiled paleo-reconstructions of Heinrich stadial 11 of the penultimate deglaciation between 135 and 130 thousand years ago, suggesting an increase in the transport of latent heat from the Southern Hemisphere to the Northern Hemisphere, causing transient warming in the Northern Hemisphere (termination II interstadial, TII IS) and an increase in Indian summer monsoon rainfall. This transient warming facilitated the Northern Hemisphere ice sheet melting, which then might have reduced or shut down the AMOC, causing cooling of the Northern Hemisphere and East Asia and a subsequent reduction of the length of the monsoon rain season (e.g., Wassenburg et al., 2021). Mechanistically, a reduction of the AMOC strength either via warming and induced ice sheet melting or increased Eurasian–Arctic river runoff (e.g., Zhang et al., 2013) cools the Northern Hemisphere and shifts the ITCZ southward (Chemke et al., 2022), affecting spatial rainfall patterns as well as the distribution and amount of rainfall in the Northern Hemisphere semi-arid and tropical monsoon regions of India and Asia.
An AMOC weakening has also been shown to strengthen the Indo-Pacific Walker circulation via cooling of the equatorial Pacific and warming of the Southern Hemisphere–Antarctic climate on a multi-decadal timescale (e.g., Orihuela-Pinto et al., 2022a). The observed AMOC weakening during the last decades might be partially affected by interannual ocean–atmosphere interactions, such as the ENSO. These superimposed effects, operating across timescales, alter relationships between the ENSO and tropical monsoons and thereby regional rainfall patterns in a warmer climate (Mahendra et al., 2021; Pandey et al., 2020). For example, while the linear effect of ENSO on the Indian summer monsoon rainfall has weakened, the effect of ENSO on the West African monsoon has increased in recent decades (Srivastava et al., 2019).
Both relationships, between ENSO and the Indian summer monsoon and also between ENSO and the West African monsoon, need to be further tested in paleoclimate reconstructions from both warm and cold climate states to gain a better understanding of how an abrupt change in AMOC may have an effect on ENSO and/or on tropical monsoon systems. This would allow for a more robust predictability of tropical monsoon rainfall patterns in the future.
2.7 Effects of permafrost regions on the global hydrological cycle
The permafrost regions have accumulated substantial amounts of ice in the soils. With ground ice melting away in a warmer climate, permafrost landscapes are experiencing drastic hydrological changes. The presence of ice modulates the thermophysical soil properties as well as infiltration rates and the vertical and lateral movement of water through the ground, which is often poorly represented in current Earth system models and therefore exhibits large inter-model differences. Hence, uncertainty exists about high-latitude regions becoming wetter or drier in the future due to soil hydrology representation in state-of-the-art Earth system models (de Vrese et al., 2023). They could either (i) turn into a wetter and cooler state with many freshwater systems and lakes, which support increasing land–atmosphere moisture recycling and cloud cover, reducing ground temperatures, or (ii) turn into a drier state as newly formed lakes could drain, with less moisture recycling supporting less cloud cover and a warmer surface (Nitzbon et al., 2020; Liljedahl et al., 2016). Which parts of the Arctic will be wetter or drier in the future is uncertain, but the differences between the potential Arctic hydroclimatic futures could be very pronounced. As recently shown by de Vrese et al. (2023), the drier and warmer permafrost state would lead to less sea ice, a reduced pole-to-Equator temperature gradient, and a weaker AMOC. The drier state has more boreal forest extended to the north, with a higher frequency and extent of forest wildfires. In comparison with the wetter state, the drier Arctic state also shifts the position of the Intertropical Convergence Zone, which results in higher precipitation in the Sahel region and potentially also in the Amazon rainforest region. Increased forest and vegetation cover in these regions would be the consequence (de Vrese et al., 2023). Therefore, shifts in permafrost hydrology could affect climate tipping elements far beyond Arctic boundaries. Permafrost changes may impact the hydrological cycle with far-reaching impacts.
2.8 Interactions between multiple tipping elements and planetary-scale cascades
Assembling the individual links mentioned before in Sect. 2.2–2.7 gives rise to the possibility of tipping cascades involving more than two elements. These could lead to large changes at the regional and even planetary scale. Plausible examples are Dangaard–Oeschger (D/O) events (Sect. 3.2.2). Another example comes from the study of the last interglacial period, for which proxies for sea ice, polar ice sheets, AMOC, boreal forest, and permafrost indicate abrupt changes (Thomas et al., 2020). Although the dating uncertainties make it difficult to determine the causal structure of a potential cascade, positive feedbacks between these TEs could explain the amplified polar temperatures and sea level rise obtained from reconstructions (+8 ∘C in Greenland and +6–9 m sea level rise compared to today) (Dutton et al., 2015; NEEM community members, 2013).
On a larger scale, tipping cascades could be responsible for driving the Earth system into completely different climatic states that have been identified in paleo-data (Westerhold et al., 2020), climate models of intermediate complexity (Lucarini and Bódai, 2017), and general circulation models at coarse spatial resolution (Brunetti et al., 2019; Popp et al., 2016; Ferreira et al., 2011; Voigt and Marotzke, 2010). For example, a tipping cascade involving ocean circulation and ice sheets might have been responsible for a transition from a greenhouse to an icehouse state at the Eocene–Oligocene boundary (Sect. 3.1).
While unlikely, a major concern regarding the future may be that a cascade involving several tipping elements and feedbacks could lock the Earth system in a pathway towards a hothouse state with conditions resembling those of the mid-Miocene (hothouse hypothesis: +4–5 ∘C, +10–60 m sea level compared to the pre-industrial baseline) (Burke et al., 2018; Steffen et al., 2018). Feedbacks that affect global temperature could involve albedo changes (e.g., through ice sheet or sea ice loss), and additional CO2 and CH4 emissions (through permafrost thawing, methane hydrates release) may lead to additional warming on medium to long timescales (Wunderling et al., 2020; Steffen et al., 2018). In a worst-case (and unlikely) scenario, it has been speculated that a regional breakup of stratocumulus decks at atmospheric CO2 levels above 1200 ppm could translate into a large-scale temperature feedback leading to a warming of roughly 8 ∘C (Schneider et al., 2019).
Timescales are crucial when discussing hothouse scenarios. A potential hothouse state in the next centuries seems implausible in light of the current state of research. For example, in climate projections up to 2100, CMIP6 (Coupled Model Intercomparison Project) GCMs show no evidence of nonlinear responses on the global scale. Instead, they show a nearly linear dependence of global mean temperature on cumulative CO2 emissions (Masson-Delmotte et al., 2021). Similarly, in a recent assessment, Wang et al. (2023) concluded that a tipping cascade with large temperature feedbacks over the next couple of centuries remains unlikely and that while the combined effect of tipping elements on temperature is significant for those timescales, it is secondary to the choice of anthropogenic emissions trajectory. However, this does not completely rule out the possibility of a hothouse scenario in the longer term. Indeed, tipping events are not necessarily abrupt on human timescales. Positive feedbacks could have negligible impacts by 2100, for example on global mean temperature and sea level rise, but still influence Earth system trajectories on (multi-)millennial timescales (Kemp et al., 2022; Lenton et al., 2019; Steffen et al., 2018). To the authors' knowledge, no multi-millennial simulation with a model incorporating the elements and feedbacks described above has yet been conducted to test this scenario. This calls for experiments across the model hierarchy. EMICs (Earth system models of intermediate complexity) in particular, and AOGCMs at coarse spatial resolution, offer an interesting trade-off as they include representations of most tipping elements while still allowing for multi-millennial simulations.
In this section, we outline tipping sequences that have been observed in the distant and less distant past. We discuss how the Eocene–Oligocene transition and interactions since the last (inter)glacial periods (Bølling–Allerød, Dansgaard–Oeschger, Heinrich events) may fit into the framework of a tipping cascade.
3.1 Interactions in the distant past: Eocene–Oligocene transition
The formation of a continent-scale ice sheet on Antarctica during the Eocene–Oligocene transition about 34 million years ago is known as Earth's greenhouse–icehouse transition (see Fig. 3). Following a cooling over tens of millions of years, this shift to a new climate state would have been visible from space, as Antarctic forests were replaced by a blanket of ice, and seawater receded from the continents, changing the shapes of coastlines worldwide. The climate transition is recorded as a shift in the oxygen isotopic composition of microscopic fossil shells in marine sediment cores, which reflects a combination of deep-sea cooling and continental ice growth (Coxall et al., 2005). It had global consequences for Earth’s flora and fauna, both in the oceans and on land (Hutchinson et al., 2021).
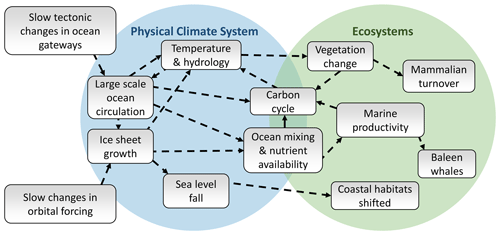
Figure 4Conceptual linkages between changes in the Earth system associated with the Eocene–Oligocene transition 34 million years ago. External drivers were the slow changes in ocean gateways caused by tectonic plate movement and slow changes in Earth's orbital configuration. The interactions and feedbacks within the Earth system act on different timescales, which makes the complete sequence of events complicated, but overall these processes resulted in Earth's greenhouse–icehouse transition. Since all links are uncertain, they are denoted with dashed lines (compare Fig. 5).
This climate transition has been identified as a possible paleoclimate example of cascading tipping points in the Earth system (Dekker et al., 2018; Tigchelaar et al., 2011). Examples of climatic tipping elements in this case consist of global deep-water formation, the Antarctic Ice Sheet, polar sea ice, monsoon systems, and tropical forests. In a conceptual model, the first part of the oxygen isotope shift is attributed to a major transition in global ocean circulation, while the second phase reflects the subsequent blanketing of Antarctica with a thick ice sheet (Tigchelaar et al., 2011).
The global ocean circulatory system was showing tentative signs of change a few million years before the climate transition, likely caused by changing ocean gateways in the North Atlantic (Coxall et al., 2018). Neodymium isotopes do suggest that a precursor to North Atlantic Deep Water reached the Southern Hemisphere close to the Eocene–Oligocene transition, perhaps signaling the onset of Atlantic Meridional Overturning Circulation (AMOC) (Via and Thomas, 2006). However, the exact timing remains uncertain and may not correlate with the onset of the oxygen isotope shift. Indeed, the first part of the isotope shift is associated with a cooling of both deep-sea temperatures and low-latitude sea surface temperatures, which therefore more likely reflects a change in radiative forcing (Kennedy et al., 2015; Lear et al., 2008). However, this does not preclude AMOC onset preconditioning the system for glaciation through heat piracy in the Southern Ocean, with the exact timing of the transition set later by a favorable orbital configuration (Coxall et al., 2005).
In general, biomes in Earth's greenhouse state reflect warmer and wetter conditions than the icehouse state of the early Oligocene, but many of these seemed to have changed gradually as climate cooled in the Eocene, making it difficult to identify vegetation tipping elements following the glaciation of Antarctica (Hutchinson et al., 2021). The mammalian fossil record, which is coupled to vegetation through diet, suggests more acute changes in the early Oligocene. The Grande Coupure (i.e., “the Big Break”), is a long-known mammalian extinction–origination event during Eocene–Oligocene times involving large-scale migrations of Asian mammals into Europe (Hooker et al., 2004). Thought to signal a combination of changing climate and floral changes, this abrupt faunal turnover might reflect crossing of an ecosystem tipping point caused by the crossing of a climate tipping point: a climate–ecology tipping cascade. Mammal extinctions seem to be particularly widespread in Afro-Arabia and linked to loss of dietary diversity (de Vries et al., 2021). This finding is consistent with the idea that biomes in this subtropical region are tippable elements (Armstrong McKay et al., 2022; Lenton et al., 2008). Other evidence of vegetation biomes having tipped includes a transition from warm–temperate to cool–temperate rainforests in southeastern Australia (Korasidis et al., 2019). Monsoon systems, sensitive to forcing and to large-scale reorganizations of the climate system, might have been important for explaining the shifts in these respective vegetation biomes. Moreover, simulations of the late Eocene climate suggest the existence of a strong monsoon-like climate over the Antarctic continent; without a major reorganization of such an atmospheric circulation regime, ice growth on Antarctica seems very unlikely (Baatsen et al., 2024, 2020).
The glaciation of Antarctica also produced a sea level fall of several tens of meters (Lear et al., 2008), causing shallow seaways to recede, turning many marine regions into continental habitats, which experienced particularly strong seasonality (Toumoulin et al., 2022). The associated reduction of the marine carbonate factory in previously submerged tropical shelf seas caused the calcite compensation depth to deepen by more than 1 km, turning enormous swathes of seafloor white as the sinking calcite shells of plankton no longer dissolved in shallow depths (Coxall et al., 2005).
In summary, Earth's greenhouse–icehouse transition was likely associated with a range of interactions between components of the Earth system that are debated as potential tipping elements. Determining the extent to which these reflect a cascading series will require a major data-modeling effort, with improved correlations between marine and terrestrial records, as well as better constraints on the rate and magnitude of change within a range of tipping elements.
3.2 Interactions during and since the last glacial period
3.2.1 Bølling–Allerød
Towards the end of the last ice age, a very prominent event is recorded in numerous geological archives. The Bølling–Allerød (B/A) started at 14.7 ka with abrupt warming in the Northern Hemisphere (with polar atmospheric circulation shifts over a few years followed by temperature increase in Greenland of around 10 ∘C in the subsequent decades – Wolff et al., 2010; Steffensen et al., 2008; Landais et al., 2005; Severinghaus and Brook, 1999) in response to a reinvigoration of the AMOC (McManus et al., 2004) and lasted until 12.9 ka. The B/A is an example of pronounced interactions between Earth system components and cascading impacts in the Earth system (Brovkin et al., 2021). At the onset of the B/A, atmospheric CO2 and CH4 concentrations rapidly increased over a few decades (Marcott et al., 2014) in response to AMOC strengthening, abrupt warming and permafrost thaw (Köhler et al., 2014), and moisture changes (e.g., Kleinen et al., 2023). This was followed by fast changes in vegetation composition (Novello et al., 2017; Fletcher et al., 2010). In the ocean, surface warming and circulation changes were propagated downward, leading to sedimentary anoxia across the North Pacific (Praetorius et al., 2015; Jaccard and Galbraith, 2012) as well as more severe hypoxia in the Cariaco Basin (Gibson and Peterson, 2014) and Arabian Sea (Reichart et al., 1998), indicating a link between climate warming and ocean deoxygenation.
The trigger for the rapid amplification of ocean circulation and the associated abrupt impacts at the B/A transition has been a focus of debate, with opinions divided between an essentially linear response to the (possibly abrupt) cessation of freshwater forcing (e.g., Liu et al., 2009) versus a nonlinear response to more gradual forcing (i.e., a tipping point, e.g., Barker and Knorr, 2021; Knorr and Lohmann, 2007).
Gradual changes observed in key climatic variables (e.g., CO2 and global temperature) during sustained periods of cold across the surface North Atlantic (as occurred prior to the B/A onset) were a persistent feature of glacial terminations throughout the last 800 kyr (e.g., Barker et al., 2019), as well as during the massive ice-rafting events of the last glacial period (known as Heinrich events). Each of these periods is thought to have been followed by the rapid resumption of ocean circulation and other events associated with the B/A (e.g., a rapid rise of CO2 and CH4).
3.2.2 Dansgaard–Oeschger events
Equally rapid as B/A and of comparable magnitude, transitions known as Dansgaard–Oeschger (D/O) events (Fig. 4) occurred repeatedly during glacial periods throughout much of the late Pleistocene (e.g., Barker et al., 2011; Hodell et al., 2023). In general, these consist of an abrupt (on the order of decades) warming from stadial to interstadial conditions, followed by gradual cooling over the course of hundreds of years to a few thousand years, before a rapid transition back to cold stadial conditions. Evidence from Greenland ice cores and North Atlantic sediments suggests that the abrupt cooling transitions (from warm interstadial to cold stadial conditions) were systematically preceded and possibly triggered by more gradual cooling across the high-latitude Northern Hemisphere (e.g., NGRIP partners; Barker et al., 2015). The abrupt transitions from stadial to interstadial conditions were also preceded by more gradual changes elsewhere (for example increasing Antarctic and deep-ocean temperatures and decreasing dustiness; Barker and Knorr, 2007), leading to the idea that both types of transitions may be predictable to some extent (Lohmann, 2019; Barker and Knorr, 2016). Each event was also paired with rapid changes in ocean circulation, terrestrial hydroclimate, atmospheric composition, and ocean oxygenation in much the same way as observed during the B/A. Thus, the occurrence and interactions among many subsystems that show abrupt changes make it plausible to consider it a cascade and are a ubiquitous and common feature of late Pleistocene climate variability.
During the abrupt warming phases of D/O cycles, an abrupt decrease in Arctic and North Atlantic sea ice cover likely contributed to the onset of convection and a rapid resurgence of a much weaker, and potentially even collapsed, AMOC (Gildor and Tziperman, 2003; Li et al., 2010). D/O-type changes in coupled climate models also feature a rapid disappearance of sea ice that precedes the abrupt AMOC strengthening (Vettoretti and Peltier, 2016; Zhang et al., 2014). Lastly, D/O could also be self-sustained oscillations as recent literature pointed out (Vettoretti et al., 2022). Thus, the D/O warmings may potentially comprise a tipping cascade (Lohmann and Ditlevsen, 2021). However, such a cascading interaction may depend on the climate background state, and it is unclear whether North Atlantic sea ice cover during the last glacial period can be considered a tipping element.
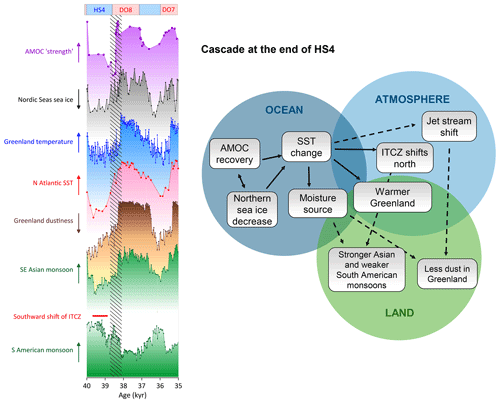
Figure 5Interactions at the end of Heinrich Stadial 4 (HS4). (a) Climate proxy indices spanning the transition from HS4 into Dansgaard–Oeschger (D/O) event 8 (time goes from left to right). From top to bottom: AMOC strength (Henry et al., 2016), Norwegian Sea ice cover (Sadatzki et al., 2020), Greenland temperature (North Greenland Ice Core Project members (NGRIP), 2004), North Atlantic SST (Martrat et al., 2007), dust accumulation in Greenland (Ruth et al., 2007), Asian monsoon intensity (Cheng et al., 2016), and South American monsoon intensity (Kanner et al., 2012). The horizontal red bar indicates the period when the ITCZ assumed a more southerly position (Wang et al., 2004). The hatched region spans the transition from HS4 to D/O8 and represents an estimate of the relative age uncertainty among the records shown (i.e., it is generally not possible to tell which changes occurred earlier or later within the overall sequence). Vertical arrows indicate the direction of increase for each parameter. (b) Interactions between the ocean, atmosphere, and land during the end of HS4. Linkages with comparably high uncertainty are denoted with dashed arrows.
3.2.3 Heinrich events
While the exact causes and mechanisms of the B/A transition and D/O events are still under debate, Heinrich events are better understood. They occurred during some of the cold stadial phases mentioned above and were associated with major reorganization of ocean circulation in the North Atlantic (for a review see Clement and Peterson, 2008). During Heinrich events, large masses of ice were released from the Laurentide Ice Sheet, leading to a dramatic freshening of the North Atlantic Ocean and enhanced suppression of deep-water formation and the AMOC.
They can be understood as a phenomenon involving two tipping elements, the Laurentide Ice Sheet and the AMOC. The ice fluxes from the Laurentide Ice Sheet have been described as a binge–purge oscillator (MacAyeal, 1993), where a period of strong ice accumulation (the binge phase) is followed by a period of rapid ice loss (the purge phase). During the binge phase, ice is generally thought to be frozen to the bottom and thus immovable. As the ice sheet gets thicker, basal temperatures increase until the pressure melting point of the basal ice is reached. The resulting meltwater production lubricates the bed and enables sliding of the ice. This may already be sufficient to initiate the purge phase, though further triggers like ocean subsurface warming probably also played an important role in destabilizing marine-terminating portions of the Laurentide Ice Sheet (Max et al., 2022; Alvarez-Solas et al., 2013). The purge phase lasts until the ice sheet has become too thin to maintain basal temperatures above the pressure melting point, thus refreezing and stopping the ice flow. The resulting ice stream flows into the Atlantic Ocean, and as the resulting icebergs melt, Atlantic surface waters are freshened to the point where the AMOC cannot be sustained and collapses.
The mechanisms sketched above have been demonstrated in a number of transient model experiments using Earth system models of intermediate complexity (Calov et al., 2010, 2002) and complex ice sheet–atmosphere–ocean general circulation models (Schannwell et al., 2023; Ziemen et al., 2019). However, not all details have yet been resolved; the exact trigger mechanism (and threshold) initiating the purge phase, for example, has not yet been identified (Schannwell et al., 2023).
3.3 A paleoclimate perspective on the resilience of the Amazon rainforest
Two historical analogs have provided some (albeit not fully consistent) insights into the response of the Amazon to reductions in rainfall: Heinrich events during the last glacial period and the mid-Holocene. As mentioned in the previous section, Heinrich events are remarkable intervals during the last glacial period in which the AMOC seems to have substantially weakened in response to iceberg release in the North Atlantic (Henry et al., 2016). Paleoclimate data from these events are of great help to evaluate the processes suggested by climate model simulations of AMOC slowdown. Häggi et al. (2017), using an isotope proxy from a sediment core collected offshore of the Amazon River mouth, showed savanna intrusions into the Amazon rainforest during repeated Heinrich events. The intrusions of savanna occurred in northern Amazonia (Zular et al., 2019; Häggi et al., 2017) and validate the suggested decrease in precipitation over that region in response to AMOC weakening (Campos et al., 2019). Further precipitation and, even more importantly, vegetation reconstructions with appropriate age models and sufficient temporal resolution will help clarify the southward extent of the drying of northern Amazonia due to an AMOC collapse, as well as its consequences for the rainforest.
Kukla et al. (2021) used pollen, charcoal, and speleothem oxygen isotope proxy data to reconstruct the response of the Amazon forest during the mid-Holocene, when precipitation was relatively low (Prado et al., 2013). Their analysis suggests that the Amazon was resilient to rainfall reductions as high as projected by climate models for the rest of the century. However, it also has to be considered that in the study of Kukla et al. (2021) temperature and land use were similar to pre-industrial conditions, whereas future warming and deforestation will act as additional stressors that affect the surface water balance by increasing potential evapotranspiration and decreasing precipitation recycling (Zemp et al., 2017), while also increasing the chances of fire and thus the possibility of the Amazon being converted into a degraded, open ecosystem.
Modeling interactions between tipping elements and potential tipping cascades in the climate system is a difficult task. A key challenge is to accurately capture feedback mechanisms between different climatic components. In addition, each climate subsystem evolves over spatial scales and timescales that can span orders of magnitude from decades to centuries for biosphere components and from centuries to millennia for the large ice sheets on Greenland and Antarctica (see Fig. 2). The ideal tool to study the interaction between tipping elements would therefore be a high-resolution, comprehensive Earth system model based on general circulation models for the atmosphere and ocean, with a sea ice component, dynamic vegetation, and interactive ice sheets and carbon cycle. Moreover, the model should be computationally fast to allow the representation of the slow processes and to run comprehensive ensembles for taking into account the uncertainties in key parameters (Murphy et al., 2004). However, such a universal tool does not exist. Instead, a hierarchy of models of different complexity is needed to explore the interactions between tipping elements on different temporal and spatial scales (Fyke et al., 2018). The state-of-the-art Earth system models that are usually employed in climate change projections, the models in the CMIP6 (Eyring et al., 2016), are the first choice to investigate processes developing over centennial timescales. Since these models usually include dynamic vegetation, they are suitable to explore the interactions between abrupt changes in ocean circulation, Arctic sea ice, and vegetation cover. However, CMIP6 GCMs are computationally expensive and most of them do not include interactive ice sheets, which limits the applicability of these models to study interactions between tipping elements and potential tipping cascades that involve slow deep-ocean dynamics or ice sheets. Also, they show some limitations to how vegetation is represented, especially in tropical areas (e.g., D’Onofrio et al., 2020). Recently, progress has been made in including interactive ice sheets in a few CMIP6 models for studying the coupled climate–Greenland evolution (Madsen et al., 2022; Ackermann et al., 2020; Muntjewerf et al., 2020) on centennial timescales.
For studying feedbacks on millennial timescales or longer, one possible solution is to use Earth system models with coarser spatial resolution, allowing for faster simulations (Brunetti and Ragon, 2023; Brunetti et al., 2019; Ferreira et al., 2011; Hawkins et al., 2011), or Earth system models of intermediate complexity (EMICs, Claussen et al., 2002). A downside of these models is that the interactions between tipping elements are necessarily less realistic and some nonlinear processes need to be parameterized at sub-grid level. In particular, EMICs are faster than GCMs of comparable spatial resolution, since they make use of some approximations in the representation of the atmosphere and/or ocean dynamics and can, for instance, be applied to investigate climate–ice sheet interactions on multi-millennial timescales (Willeit et al., 2022; Quiquet et al., 2021; Choudhury et al., 2020).
An alternative technique to speed up complex models, and therefore enable them to explore feedbacks on longer timescales, is offline (asynchronous) coupling, which has been applied to represent vegetation–climate (Betts et al., 1997; De Noblet et al., 1996; Claussen, 1994) and ice sheet–climate interactions (Scherrenberg et al., 2023; Pohl et al., 2016; Herrington and Poulsen, 2011; Pollard, 2010). One example of asynchronous coupling is when the atmosphere evolves with fixed vegetation cover and eventually the latter is updated to the equilibrium conditions of the former (Foley et al., 1998). However, despite being less computationally expensive, feedback mechanisms and thus tipping phenomena and cascades are better represented when dynamical (synchronous) coupling is implemented (Drüke et al., 2021; Fisher et al., 2018; Fyke et al., 2018; Bonan et al., 2003).
It is important to note that such complex process-based models with sufficient integration of tipping elements (e.g., in EMICs or GCMs) are now starting to become available, for instance when ice sheet models are dynamically integrated in Earth system models or when new versions of EMICs are developed (Pöppelmeier et al., 2023; Willeit et al., 2022; Kreuzer et al., 2021). This will open up new possibilities for the simulation of cascading transitions.
Tipping phenomena and cascades at the regional scale, however, may be investigated with different approaches (Bastiaansen et al., 2022). For instance, they can be investigated using observation-based simulations coupled to energy or hydrological balance models at high spatial resolution as has been done for tipping cascades in the Amazon rainforest (Wunderling et al., 2022). Regional implementations of ice shelf–ocean interaction exist to obtain improved estimates of basal melt and to include small-scale processes, like the presence of ocean eddies (Dinniman et al., 2016) that can affect the overall stability of the system and potentially intensify transitions or cascades. It is indeed possible to run regional climate models (RCMs) (Noël et al., 2018; Rae et al., 2012) at horizontal grid resolutions of a few kilometers, thus providing more accurate spatiotemporal distributions of climatic variables like precipitation and temperature than GCMs. An alternative modeling framework is to apply grid refinement over a specified region of interest in a GCM, which avoids inconsistencies between the different dynamical cores and physical parameterizations used in RCMs and GCMs, and (more importantly for tipping phenomena or cascades) it allows for two-way interactions between the refinement region and the global domain (van Kampenhout et al., 2019). With such regionalized modeling approaches it could be possible to empirically detect local to super-regional (cascading) regime shifts (Rocha et al., 2018).
Also, more conceptual approaches based on differential equations or box models are frequently used for studying tipping events and cascades for present-day climate and paleoclimates (Lohmann et al., 2021; Wunderling et al., 2021b; Wood et al., 2019; Boers et al., 2018). While such models offer a unique way of unraveling the complex dynamics of interacting tipping elements, it is not guaranteed that results of conceptual models can be confirmed by complex models, since the former consider only a limited subset of dynamical variables and nonlinear processes of the climate system (Bathiany et al., 2016). For example, simple models that do not include space are suggested to overpredict the existence of tipping points, while spatial pattern formation phenomena might prevent such tipping when space is explicitly included (Rietkerk et al., 2021).
Lastly, modeling of cascading effects from the physical system to society and the economy as well as vice versa is still missing from most state-of-the-art Earth system models, requiring urgent development (Franzke et al., 2022; Steffen, 2021; Beckage et al., 2020)
As anthropogenic global warming continues, tipping elements are at risk of crossing critical thresholds (Armstrong McKay et al., 2022). Several assessments have investigated the risk of crossing critical thresholds of individual tipping elements, whereas interactions between tipping elements have only more recently been taken into account, mostly by conceptual models (e.g., Sinet et al., 2023; Wunderling et al., 2023; Dekker et al., 2018). In this review, we summarize the current state of the literature of many central tipping element interactions. Tipping elements interact across scales in space and time (see Figs. 1 and 2), spanning from sub-continental to nearly planetary spatial scales from sub-yearly up to millennial timescales. We summarize the discussed interactions between tipping elements in Table 1. Altogether, we conclude the following.
-
Out of the discussed interactions in this review, 13 are assessed as destabilizing, while 2 are stabilizing and 4 are of unclear sign (see Figs. 1 and 3). This means that the majority of interactions are destabilizing. While confirmation or rejection through future research is necessary, the possibility cannot be ruled out that interactions between climate tipping elements destabilize the Earth system in addition to climate change effects on individual tipping elements. Therefore, tipping cascades cannot be ruled out when tipping thresholds of the first tipping elements are transgressed through ongoing global warming.
-
The core tipping elements with the lowest thresholds are the large ice sheets on Greenland and West Antarctica. They have been suggested as the initiators of tipping cascades (Wunderling et al., 2021a). However, those are also the tipping elements with the largest tipping time (several centuries up to millennia). Thus, if their tipping points are only transgressed for a limited amount of time (overshoot), cascading tipping risks are also reduced (Wunderling et al., 2023, see the Supplement).
-
However, if global temperatures reach global warming levels beyond 2.0 ∘C (or stay between 1.5 and 2.0 ∘C for a centennial timescale), more and more fast tipping elements like the AMOC or the Amazon rainforest would also be at risk of tipping and could then initiate a cascading transition on a faster timescale (Wunderling et al., 2023, see the Supplement).
As such, our results are in line with earlier assessments, in particular on timescales of tipping element interactions and possible cascading transitions, but also the current state of our knowledge gaps for tipping cascade risks (Wang et al., 2023). Summarized, much uncertainty remains and most studies as of now rely on conceptual models rather than detailed process-based Earth system models. Further, it is important to point out that most of the agreement and evidence in Table 1 refer to interactions in the context of natural climate variability or gradual forced climate change. To what extent the climate system can be considered nonlinear enough to produce tipping points, but on the other hand linear enough to extrapolate these interactions from small amplitudes to extreme changes related to tipping, needs to be tested. Therefore, assessing the overall stability of the Earth system, and the possibility of a chain of nonlinear transitions, will require more detailed assessments of interactions, their effect strengths, timescales, and state dependence.
While there is more and more research on individual thresholds of climate tipping elements, substantial uncertainties prevail in the existence and strength of many links between tipping elements. In order to decrease such uncertainties, we propose four possible ways forward. (i) Observation-based approaches: satellite observations, reanalysis, and paleoclimate data sets may be evaluated using correlation measures (Liu et al., 2023) or more advanced causal inference approaches (e.g., Runge et al., 2019a; Kretschmer et al., 2016; Runge et al., 2015; Van Nes et al., 2015). (ii) Approaches based on Earth system models: with recent progress, Earth system models of full or intermediate complexity could be used to evaluate interactions between climate tipping elements in process detail and quantify their interactions using specifically designed experiments. (iii) Risk analysis approaches: since relevant parameter and structural uncertainties are large within Earth system models, analyzing model ensembles with a considerable number of ensemble members is very helpful in order to comprehensively propagate uncertainties for risk assessments (Daron and Stainforth, 2013; Stainforth et al., 2007; Murphy et al., 2004). While this approach often requires more simplified or emulator models designed for large-scale Monte Carlo analyses, it does not reduce model or data uncertainties per se. Therefore, it is still possible to evaluate the risk of emerging tipping events or cascades as well as the role of interactions between tipping elements. (iv) Expert elicitation: an expert elicitation on tipping element interactions would be valuable to update and move beyond early investigations of this kind (Kriegler et al., 2009), since all the three aforementioned approaches (i)–(iii) have important limitations that would benefit from direct expert input.
Clearly all of these strategies have their strengths and limitations. Both (i) and (ii) could benefit from extending the established notion of correlation measures in climate networks (Liu et al., 2023; Ciemer et al., 2021; Armstrong et al., 2019; Svendsen et al., 2014; Chen et al., 2010) to causal measures such as causal inference methods, for instance informed by Granger causality or conditional (in)dependences (Pearl, 1985; Granger, 1969). A prominent approach for causal inference has been applied in climate science using the so-called PCMCI algorithm (Runge et al., 2019b), which is a constraint-based method that considers (lagged) partial correlation to establish links between the considered variables. Such methods can be used to check whether identified correlations indeed correspond to a causal relation, where it is important to take into account all possible confounding factors. To do that one needs to start from the physical processes involved, for instance informed by conceptual models, and test this as a hypothesis for the causal relations (Kretschmer et al., 2021; Di Capua et al., 2020). Another example of such a causal method is the Liang–Kleeman information flow method, which is based on the rate of information transfer in dynamical systems and has been rigorously derived from the propagation of information entropy between variables (Liang, 2021; Liang and Kleeman, 2005). The method has been recently applied to Earth system processes, including Antarctic surface mass balance (Vannitsem et al., 2019) and Arctic sea ice (Docquier et al., 2022). However, to apply such methods to the tipping point context it is important to know the limitations. One of the assumptions made is that of stationarity of the links between the variables considered, which may not be true once a tipping point is crossed. Another difficulty may be the different timescales of the tipping elements where, e.g., ice sheets are very slow compared to the Amazon rainforest or AMOC.
Limitations of the approaches (i)–(iv) further include the following. First, data from recent Earth observation efforts (e.g., for AMOC by RAPID and OSNAP – Srokosz and Bryden, 2015 – or for ice stream velocities by satellite laser altimetry from ICESat/ICESat-2 – Abdalati et al., 2010; Schutz et al., 2005) may need to be extended to cover more variables relevant to Earth system tipping elements as well as better covering the relevant temporal and spatial scales. Paleoclimate data can partially compensate for such disadvantages at the cost of the data being hard to retrieve with availability and abundance far from perfect. Second, complex Earth system models may not include all relevant interaction processes between tipping elements or are often computationally too expensive to run large-scale ensembles that could take into account and propagate all relevant uncertainties. However, kilometer-scale models are under development and tipping point simulations on this scale will likely become feasible within the next decade due to current developments and further growing computational resources (Hewitt et al., 2022; Slingo et al., 2022). And third, risk analysis approaches include accounting for theoretical knowledge properties of these types of physical dynamical systems. Therefore, different approaches should complement each other, requiring experts to combine observations, reconstructions, and novel computational strategies individually, but also potentially through a formalized elicitation. Taken together, all approaches mentioned above are required to obtain more reliable estimates of existential risks such as those posed by potential tipping events or even cascades (Kemp et al., 2022; Jehn et al., 2021). They could be used to inform an emulator for tipping risks taking into account properties of individual tipping elements as well as their interactions. In addition, there are also large uncertainties among the known interactions as discussed above, and also not all interactions are known or quantified (known unknowns versus unknown unknowns).
Further, in certain systems, there are forcings of non-climatic origin that could interact with climate change and lead to tipping and thus to interactions and possibly cascades with other elements. For instance, land use change and, specifically, deforestation are threatening the Amazon and decreasing its resilience to climate change (e.g., Staal et al., 2020), since the Amazon is transpiring large parts of its own rainfall. Recent studies also indicate that the Amazon and other humid forests might also affect the atmospheric convergence of moisture (Makarieva et al., 2023), which might possibly affect other climate and ecosystem elements. Therefore, non-climate-related factors might also trigger cascading tipping, which would require further research to be investigated. Lastly, systems do not necessarily tip fully in one go, but there can also be stable intermediate states (such as through the formation of spatial patterns). This has mostly been reported in ecological systems but is not limited to them (Rietkerk et al., 2021; Bastiaansen et al., 2020).
Taken together, assessing and quantifying tipping element interactions better have great potential to advance suitable risk analysis methodologies for climate tipping events and cascades, especially because it is clear that tipping elements are not isolated systems and appropriate risk assessment tools are yet to be developed.
The data sets used in Fig. 5 can be found in the following references for (top to bottom) AMOC strength (Henry et al., 2016), Norwegian Sea ice cover (Sadatzki et al., 2020), Greenland temperature (North Greenland Ice Core Project members (NGRIP), 2004), North Atlantic SST (Martrat et al., 2007), dust accumulation in Greenland (Ruth et al., 2007), Asian monsoon intensity (Cheng et al., 2016), and South American monsoon intensity (Kanner et al., 2012).
The supplement related to this article is available online at: https://doi.org/10.5194/esd-15-41-2024-supplement.
NW and AvdH designed the study. NW and ASvdH led the writing of the paper with input from all authors. NW, ASvdH, NJS, YA, SB, JTB, VB, TK, RW, VC, AK, HC, and CL designed the figures of this paper. All authors have reviewed and edited the final version of the paper.
At least one of the (co-)authors is a member of the editorial board of Earth System Dynamics. The peer-review process was guided by an independent editor, and the authors also have no other competing interests to declare.
For the EU projects the work reflects only the authors' view; the European Commission and their executive agency are not responsible for any use that may be made of the information the work contains.
Publisher's note: Copernicus Publications remains neutral with regard to jurisdictional claims made in the text, published maps, institutional affiliations, or any other geographical representation in this paper. While Copernicus Publications makes every effort to include appropriate place names, the final responsibility lies with the authors.
This article is part of the special issue “Tipping points in the Anthropocene”. It is a result of the “Tipping Points: From Climate Crisis to Positive Transformation” international conference hosted by the Global Systems Institute (GSI) and University of Exeter (12–14 September 2022), as well as the associated creation of a Tipping Points Research Alliance by GSI and the Potsdam Institute for Climate Research, Exeter, Great Britain, 12–14 September 2022.
This review article has been carried out within the framework of the Global Tipping Points Report 2023. We thank Timothy Lenton, David Armstrong McKay, and Sina Loriani for many fruitful discussions within this framework. Nico Wunderling and Jonathan F. Donges acknowledge support from the European Research Council advanced grant project ERA (Earth Resilience in the Anthropocene, ERC-2016-ADG-743080). Jonathan F. Donges is grateful for financial support by the project CHANGES funded by the German Federal Ministry for Education and Research (BMBF) within the framework “PIK_Change” under grant 01LS2001A. Ricarda Winkelmann acknowledges financial support via the Earth Commission, hosted by FutureEarth. The Earth Commission is the science component of the Global Commons Alliance, a sponsored project of Rockefeller Philanthropy Advisors, with support from Oak Foundation, MAVA, Porticus, Gordon and Betty Moore Foundation, Herlin Foundation, and the Global Environment Facility. The Earth Commission is also supported by the Global Challenges Foundation. Victor Couplet is funded as a research fellow by the Belgian National Fund of Scientific Research (F.S.R. – FNRS). Ann Kristin Klose and Ricarda Winkelmann acknowledge support by the European Union's Horizon 2020 research and innovation program under grant agreement no. 820575 (TiPACCs) and no. 869304 (PROTECT). Thomas Kleinen acknowledges support through the project PalMod, funded by the German Federal Ministry of Education and Research (BMBF), under grant no. 01LP1921A. Maura Brunetti acknowledges financial support from the Swiss National Science Foundation (Sinergia project no. CRSII5_180253). Coastal and marine sections would like to acknowledge the generous support of the BNP PARIBAS Foundation for the CORESCAM project, part of the 2019 call on Biodiversity and Climate Change, and the USAID for support for the SWAMP project. John T. Bruun gratefully acknowledges the UK Research Councils funded Models2Decisions grant (M2DPP035: EP/P0167741/1), ReCICLE (NE/M004120/1), and STFC Spark Award (ST/V005898/1), which helped fund his involvement with this work. Anna von der Heydt and Swinda K. J. Falkena acknowledge funding by the Dutch Research Council (NWO) under a Vici project to Anna von der Heydt (with project number VI.C.202.081 of the NWO Talent program). Anna von der Heydt has worked under the program of the Netherlands Earth System Science Centre (NESSC), financially supported by the Ministry of Education, Culture and Science (OCW). Anna von der Heydt, Robbin Bastiaansen, and Sacha Sinet acknowledge funding from the European Union's Horizon 2020 research and innovation program under grant agreement no. 820970 (this paper is TiPES paper no. 232) and under the Marie Skłodowska-Curie grant agreement no. 956170 (CriticalEarth). Caroline H. Lear acknowledges NERC funding for SWEET grant NE/P019102/1. Jonathan Barichivich acknowledges funding by the European Research Council (ERC) under the Horizon Europe research and innovation program (ERC-starting grant CATES, grant agreement no. 101043214). Cristiano M. Chiessi acknowledges financial support from FAPESP (grants 2018/15123-4 and 2019/24349-9) and CNPq (grant 312458/2020-7). Yevgeny Aksenov and Stefanie Rynders acknowledge support from the following projects: COMFORT (grant agreement no. 820989) under the European Union's Horizon 2020 research and innovation program; the EC Horizon Europe project OptimESM “Optimal High Resolution Earth System Models for Exploring Future Climate Changes” under grant 101081193 and UKRI grant 10039429; EPOC, EU grant 101059547; UKRI grant 10038003; and the UK NERC projects LTS-M BIOPOLE (NE/W004933/1), CANARI (NE/W004984/1), and Consequences of Arctic Warming for European Climate and Extreme Weather (ArctiCONNECT, NE/V004875/1). Yevgeny Aksenov and Stefanie Rynders acknowledge the use of the ARCHER UK National Supercomputing and JASMIN. David Docquier is funded by the Belgian Science Policy Office (BELSPO) under the RESIST project (contract no. RT/23/RESIST). Mara Baudena acknowledges the Italian National Biodiversity Future Center (NBFC) – National Recovery and Resilience Plan (NRRP), Mission 4 Component 2, Investment 1.4 of the Italian Ministry of University and Research, funded by the European Union – NextGenerationEU (project code CN_00000033). Matteo Willeit acknowledges financial support by the German climate modeling project PalMod supported by the German Federal Ministry of Education and Research (BMBF) as a Research for Sustainability initiative (FONA) (grant nos. 01LP1920B and 01LP1917D). Didier Swingedouw acknowledges financial support by the RRI “Tackling Global Change” from the University of Bordeaux and by the INSU/LEFE DECORATING and the UKRI DECADAL projects.
This research has been supported by the European Research Council, H2020 European Research Council (grant nos. 743080, 820575, 869304, 820970, 101043214, 820989, and 101059547), the Bundesministerium für Bildung und Forschung (grant nos. 01LS2001A, 01LP1921A, 01LP1920B, and 01LP1917D), the Natural Environment Research Council (grant nos. NE/P019102/1, NE/W004933/1, NE/W004984/1, and NE/V004875/1), the Swiss National Science Foundation (grant no. CRSII5_180253), Research Councils UK (grant nos. EP/P0167741/1, NE/M004120/1, and ST/V005898/1), the Nederlandse Organisatie voor Wetenschappelijk Onderzoek (grant no. VI.C.202.081), the Fondation BNP Paribas (grant no. CORESCAM), the United States Agency for International Development (grant no. SWAMP), the Netherlands Earth System Science Centre (grant no. NESSC), the HORIZON EUROPE Marie Skłodowska-Curie Actions (grant no. 956170), the Fundação de Amparo à Pesquisa do Estado de São Paulo (grant nos. 2018/15123-4 and 2019/24349-9), the Conselho Nacional de Desenvolvimento Científico e Tecnológico (grant no. 312458/2020-7), UK Research and Innovation (grant no. 10039429), and the Belgian Federal Science Policy Office (grant no. RT/23/RESIST). This work was supported by the TipESM project funded by the European Union's Horizon Europe research and innovation program under grant agreement no. 101137673 as well as the Swedish Research Council (DNR 2008-2859).
This paper was edited by Jesse Abrams and reviewed by B. van der Bolt, Steven Lade, and two anonymous referees.
Abdalati, W., Zwally, H. J., Bindschadler, R., Csatho, B., Farrell, S. L., Fricker, H. A., Harding, D., Kwok, R., Lefsky, M., Markus, T., Marshak, A., Neumann, T., Palm, S., Schutz, B., Smith, B., Spinhirne, J., and Webb, C.: The ICESat-2 laser altimetry mission, Proc. IEEE, 98, 735–751, 2010. a
Ackermann, L., Danek, C., Gierz, P., and Lohmann, G.: AMOC Recovery in a multicentennial scenario using a coupled atmosphere-ocean-ice sheet model, Geophys. Res. Lett., 47, e2019GL086810, https://doi.org/10.1029/2019GL086810, 2020. a, b
Alvarez-Solas, J., Robinson, A., Montoya, M., and Ritz, C.: Iceberg discharges of the last glacial period driven by oceanic circulation changes, P. Natl. Acad. Sci. USA, 110, 16350–16354, 2013. a
Aragão, L. E.: The rainforest's water pump, Nature, 489, 217–218, 2012. a
Armstrong, E., Valdes, P., House, J., and Singarayer, J.: Investigating the feedbacks between CO2, vegetation and the AMOC in a coupled climate model, Clim. Dynam., 53, 2485–2500, 2019. a
Armstrong McKay, D. I., Staal, A., Abrams, J. F., Winkelmann, R., Sakschewski, B., Loriani, S., Fetzer, I., Cornell, S. E., Rockström, J., and Lenton, T. M.: Exceeding 1.5 C global warming could trigger multiple climate tipping points, Science, 377, eabn7950, https://doi.org/10.1126/science.abn7950, 2022. a, b, c, d, e, f, g, h
Årthun, M., Eldevik, T., Smedsrud, L., Skagseth, Ø., and Ingvaldsen, R.: Quantifying the influence of Atlantic heat on Barents Sea ice variability and retreat, J. Clim., 25, 4736–4743, 2012. a
Ayarzagüena, B., Ineson, S., Dunstone, N. J., Baldwin, M. P., and Scaife, A. A.: Intraseasonal effects of el niño–southern oscillation on North Atlantic climate, J. Clim., 31, 8861–8873, 2018. a, b
Baatsen, M., von der Heydt, A. S., Huber, M., Kliphuis, M. A., Bijl, P. K., Sluijs, A., and Dijkstra, H. A.: The middle to late Eocene greenhouse climate modelled using the CESM 1.0.5, Clim. Past, 16, 2573–2597, https://doi.org/10.5194/cp-16-2573-2020, 2020. a
Baatsen, M., Bijl, P., von der Heydt, A., Sluijs, A., and Dijkstra, H.: Resilient Antarctic monsoonal climate prevented ice growth during the Eocene, Clim. Past, 20, 77–90, https://doi.org/10.5194/cp-20-77-2024, 2024. a
Bakker, P., Schmittner, A., Lenaerts, J., Abe-Ouchi, A., Bi, D., van den Broeke, M., Chan, W.-L., Hu, A., Beadling, R., Marsland, S., Mernhild, S., Saenko, O., Swingedouw, D., Sullivan, A., and Yin, J.: Fate of the Atlantic Meridional Overturning Circulation: Strong decline under continued warming and Greenland melting, Geophys. Res. Lett., 43, 12–252, 2016. a
Barbante, C., Barnola, J.-M., Becagli, S., Beer, J., Bigler, M., Boutron, C., Blunier, T., Castellano, E., Cattani, O., Chappellaz, J., Dahl-Jensen, D., Debret, M., Delmonte, B., Dick, D., Falourd, S., Faria, S., Federer, U., Fischer, H., Freitag, J., Frenzel, A., Fritzsche, D., Fundel, F., Gabrielli, P., Gaspari, V., Gersonde, R., Graf, W., Grigoriev, D., Hamann, I., Hansson, M., Hoffmann, G., Hutterli, M. A., Huybrechts, P., Isaksson, E., Johnsen, S., Jouzel, J., Kaczmarska, M., Karlin, T., Kaufmann, P., Kipfstuhl, S., Kohno, M., Lambert, F., Lambrecht, A., Lambrecht, A., Landais, A., Lawer, G., Leuenberger, M., Littot, G., Loulergue, L., Lüthi, D., Maggi, V., Marino, F., Masson-Delmotte, V., Meyer, H., Miller, H., Mulvaney, R., Narcisi, B., Oerlemans, J., Oerter, H., Parrenin, F., Petit, J.-R., Raisbeck, G. amd Raynaud, D., Röthlisberger, R., Ruth, U., Rybak, O., Severi, M., Schmitt, J., Schwander, J., Siegenthaler, U., Siggaard-Andersen, M.-L., Spahni, R., Steffensen, J. P., Stenni, B., Stocker, T. F., Tison, J.-L., Traversi, R., Udisti, R., Valero-Delgado, F., van den Broeke, M. R., van de Wal, R. S. W., Wagenbach, D., Wegner, A., Weiler, K., Wilhelms, F., Winther, J.-G., and Wolff, E.: One-to-one coupling of glacial climate variability in Greenland and Antarctica, Nature, 444, 195–198, 2006. a
Barker, S. and Knorr, G.: Antarctic climate signature in the Greenland ice core record, P. Natl. Acad. Sci. USA, 104, 17278–17282, 2007. a
Barker, S. and Knorr, G.: A paleo-perspective on the AMOC as a tipping element, PAGES Magazine, 24, 14–15, 2016. a
Barker, S. and Knorr, G.: Millennial scale feedbacks determine the shape and rapidity of glacial termination, Nat. Commun., 12, 2273, https://doi.org/10.1038/s41467-021-22388-6, 2021. a
Barker, S., Knorr, G., Edwards, R. L., Parrenin, F., Putnam, A. E., Skinner, L. C., Wolff, E., and Ziegler, M.: 800,000 years of abrupt climate variability, Science, 334, 347–351, 2011. a
Barker, S., Chen, J., Gong, X., Jonkers, L., Knorr, G., and Thornalley, D.: Icebergs not the trigger for North Atlantic cold events, Nature, 520, 333–336, 2015. a
Barker, S., Knorr, G., Conn, S., Lordsmith, S., Newman, D., and Thornalley, D.: Early interglacial legacy of deglacial climate instability, Paleoceanogr. Paleocl., 34, 1455–1475, 2019. a
Bastiaansen, R., Doelman, A., Eppinga, M. B., and Rietkerk, M.: The effect of climate change on the resilience of ecosystems with adaptive spatial pattern formation, Ecol. Lett., 23, 414–429, 2020. a
Bastiaansen, R., Dijkstra, H. A., and von der Heydt, A. S.: Fragmented tipping in a spatially heterogeneous world, Environ. Res. Lett., 17, 045006, https://doi.org/10.1088/1748-9326/ac59a8, 2022. a
Bathiany, S., Dijkstra, H., Crucifix, M., Dakos, V., Brovkin, V., Williamson, M. S., Lenton, T. M., and Scheffer, M.: Beyond bifurcation: using complex models to understand and predict abrupt climate change, Dynam. Stat. Clim. Syst., 1, 1–31, 2016. a, b
Baudena, M., Tuinenburg, O. A., Ferdinand, P. A., and Staal, A.: Effects of land-use change in the Amazon on precipitation are likely underestimated, Glob. Change Biol., 27, 5580–5587, 2021. a
Beckage, B., Lacasse, K., Winter, J. M., Gross, L. J., Fefferman, N., Hoffman, F. M., Metcalf, S. S., Franck, T., Carr, E., Zia, A., and Kinzig, A.: The Earth has humans, so why don’t our climate models?, Climatic Change, 163, 181–188, 2020. a
Bel, G., Hagberg, A., and Meron, E.: Gradual regime shifts in spatially extended ecosystems, Theor. Ecol., 5, 591–604, 2012. a
Bellomo, K., Meccia, V. L., D’Agostino, R., Fabiano, F., Larson, S. M., von Hardenberg, J., and Corti, S.: Impacts of a weakened AMOC on precipitation over the Euro-Atlantic region in the EC-Earth3 climate model, Clim. Dynam., 1–20, https://doi.org/10.1007/s00382-023-06754-2, 2023. a, b, c, d
Ben-Yami, M., Good, P., Jackson, L. C., Crucifix, M., Hu, A., Saenko, O. A., Swingedouw, D., and Boers, N.: Robust and irreversible impacts of an AMOC collapse on tropical monsoon systems: a multi-model comparison, Authorea Preprints, in review, https://doi.org/10.22541/essoar.169447451.12077946/v1, 2024. a
Berk, J. v. d., Drijfhout, S., and Hazeleger, W.: Circulation adjustment in the Arctic and Atlantic in response to Greenland and Antarctic mass loss, Clim. Dynam., 57, 1689–1707, 2021. a
Betts, R. A., Cox, P. M., Lee, S. E., and Woodward, F. I.: Contrasting physiological and structural vegetation feedbacks in climate change simulations, Nature, 387, 796–799, 1997. a
Bintanja, R., van Oldenborgh, G. J., Drijfhout, S., Wouters, B., and Katsman, C.: Important role for ocean warming and increased ice-shelf melt in Antarctic sea-ice expansion, Nat. Geosci., 6, 376–379, 2013. a
Bischoff, T. and Schneider, T.: Energetic constraints on the position of the intertropical convergence zone, J. Clim., 27, 4937–4951, 2014. a
Boers, N., Ghil, M., and Rousseau, D.-D.: Ocean circulation, ice shelf, and sea ice interactions explain Dansgaard–Oeschger cycles, P. Natl. Acad. Sci. USA, 115, E11005–E11014, https://doi.org/10.1073/pnas.1802573115, 2018. a
Bonan, G. B., Levis, S., Sitch, S., Vertenstein, M., and Oleson, K. W.: A dynamic global vegetation model for use with climate models: concepts and description of simulated vegetation dynamics, Glob. Change Biol., 9, 1543–1566, 2003. a
Born, A. and Stocker, T. F.: Two stable equilibria of the Atlantic subpolar gyre, J. Phys. Oceanogr., 44, 246–264, 2014. a
Bozbiyik, A., Steinacher, M., Joos, F., Stocker, T. F., and Menviel, L.: Fingerprints of changes in the terrestrial carbon cycle in response to large reorganizations in ocean circulation, Clim. Past, 7, 319–338, https://doi.org/10.5194/cp-7-319-2011, 2011. a
Brönnimann, S., Xoplaki, E., Casty, C., Pauling, A., and Luterbacher, J.: ENSO influence on Europe during the last centuries, Clim. Dynam., 28, 181–197, 2007. a
Brovkin, V., Brook, E., Williams, J. W., Bathiany, S., Lenton, T. M., Barton, M., DeConto, R. M., Donges, J. F., Ganopolski, A., McManus, J., Praetorius, S., de Vernal, A., Abe-Ouchi, A., Cheng, H., Claussen, M., Crucifix, M., Gallopín, G., Iglesias, V., Kaufman, D. S., Kleinen, T., Lambert, F., van der Leeuw, S., Liddy, H., Loutre, M.-F., McGee, D., Rehfeld, K., Rhodes, R., Seddon, A. W., Trauth, M. H., Vanderveken, L., and Yu, Z.: Past abrupt changes, tipping points and cascading impacts in the Earth system, Nat. Geosci.e, 14, 550–558, 2021. a
Brunetti, M. and Ragon, C.: Attractors and bifurcation diagrams in complex climate models, Phys. Rev. E, 107, 054214, https://doi.org/10.1103/PhysRevE.107.054214, 2023. a
Brunetti, M., Kasparian, J., and Vérard, C.: Co-existing climate attractors in a coupled aquaplanet, Clim. Dynam., 53, 6293–6308, 2019. a, b
Buckley, M. W. and Marshall, J.: Observations, inferences, and mechanisms of the Atlantic Meridional Overturning Circulation: A review, Rev. Geophys., 54, 5–63, 2016. a
Burke, K. D., Williams, J. W., Chandler, M. A., Haywood, A. M., Lunt, D. J., and Otto-Bliesner, B. L.: Pliocene and Eocene provide best analogs for near-future climates, P. Natl. Acad. Sci. USA, 115, 13288–13293, 2018. a
Cai, W., Borlace, S., Lengaigne, M., Van Rensch, P., Collins, M., Vecchi, G., Timmermann, A., Santoso, A., McPhaden, M. J., Wu, L., England, M. H., Wang, G., Guilyardi, E., and Jin, F.-F.: Increasing frequency of extreme El Niño events due to greenhouse warming, Nat. Clim. Change, 4, 111–116, 2014. a
Cai, W., Santoso, A., Wang, G., Yeh, S.-W., An, S.-I., Cobb, K. M., Collins, M., Guilyardi, E., Jin, F.-F., Kug, J.-S., Lengaigne, M., McPhaden, M. J., Takahashi, K., Timmermann, A., Vecchi, G., Watanabe, M., and Wu, L.: ENSO and greenhouse warming, Nat. Clim. Change, 5, 849–859, 2015. a
Cai, W., Santoso, A., Collins, M., Dewitte, B., Karamperidou, C., Kug, J.-S., Lengaigne, M., McPhaden, M. J., Stuecker, M. F., Taschetto, A. S., Timmermann, A., Wu, L., Yeh, S.-W., Wang, G., Ng, B., Jia, F., Yang, Y., Ying, J., Zheng, X.-T., Bayr, T., Brown, J. R., Capotondi, A., Cobb, K. M., Gan, B., Geng, T., Ham, Y.-G., Jin, F.-F., Jo, H.-S., Li, X., Lin, X., McGregor, S., Park, J.-H., Stein, K., Yang, K., Zhang, L., and Zhong, W.: Changing El Niño–Southern oscillation in a warming climate, Nat. Rev. Earth Environ., 2, 628–644, 2021. a, b
Calov, R., Ganopolski, A., Petoukhov, V., Claussen, M., and Greve, R.: Large-scale instabilities of the Laurentide ice sheet simulated in a fully coupled climate-system model, Geophys. Res. Lett., 29, 69–1, 2002. a
Calov, R., Greve, R., Abe-Ouchi, A., Bueler, E., Huybrechts, P., Johnson, J. V., Pattyn, F., Pollard, D., Ritz, C., Saito, F., and Tarasov, L.: Results from the Ice-Sheet Model Intercomparison Project–Heinrich Event INtercOmparison (ISMIP HEINO), J. Glaciol., 56, 371–383, 2010. a
Campos, M. C., Chiessi, C. M., Prange, M., Mulitza, S., Kuhnert, H., Paul, A., Venancio, I. M., Albuquerque, A. L. S., Cruz, F. W., and Bahr, A.: A new mechanism for millennial scale positive precipitation anomalies over tropical South America, Quaternary Sci. Rev., 225, 105990, https://doi.org/10.1016/j.quascirev.2019.105990, 2019. a, b
Casas-Prat, M. and Wang, X. L.: Projections of extreme ocean waves in the Arctic and potential implications for coastal inundation and erosion, J. Geophys. Res.-Ocean., 125, e2019JC015745, https://doi.org/10.1029/2019JC015745, 2020. a, b
Chemison, A., Defrance, D., Ramstein, G., and Caminade, C.: Impact of an acceleration of ice sheet melting on monsoon systems, Earth Syst. Dynam., 13, 1259–1287, https://doi.org/10.5194/esd-13-1259-2022, 2022. a
Chemke, R., Ming, Y., and Yuval, J.: The intensification of winter mid-latitude storm tracks in the Southern Hemisphere, Nat. Clim. Change, 12, 553–557, 2022. a
Chen, W., Dong, B., and Lu, R.: Impact of the Atlantic Ocean on the multidecadal fluctuation of El Niño–Southern Oscillation–South Asian monsoon relationship in a coupled general circulation model, J. Geophys. Res.-Atmos., 115, 1–12, https://doi.org/10.1029/2009JD013596, 2010. a
Cheng, H., Edwards, R. L., Sinha, A., Spötl, C., Yi, L., Chen, S., Kelly, M., Kathayat, G., Wang, X., Li, X., Kong, X., Wang, Y., Ning, Y., and Zhang, H.: The Asian monsoon over the past 640,000 years and ice age terminations, Nature, 534, 640–646, 2016. a
Choudhury, D., Timmermann, A., Schloesser, F., Heinemann, M., and Pollard, D.: Simulating Marine Isotope Stage 7 with a coupled climate–ice sheet model, Clim. Past, 16, 2183–2201, https://doi.org/10.5194/cp-16-2183-2020, 2020. a
Ciemer, C., Winkelmann, R., Kurths, J., and Boers, N.: Impact of an AMOC weakening on the stability of the southern Amazon rainforest, Europ. Phys. J.-Spec. Top., 230, 3065––3073, 2021. a, b
Claussen, M.: On coupling global biome models with climate models, Clim. Res., 4, 203–221, 1994. a
Claussen, M., Mysak, L., Weaver, A., Crucifix, M., Fichefet, T., Loutre, M.-F., Weber, S., Alcamo, J., Alexeev, V., Berger, A., Calov, R., Ganopolski, A., Goosse, H., Lohmann, G., Lunkeit, F., Mokhov, I., Petoukhov, V., Stone, P., and Wang, Z.: Earth system models of intermediate complexity: closing the gap in the spectrum of climate system models, Clim. Dynam., 18, 579–586, 2002. a
Clemens, S. C., Yamamoto, M., Thirumalai, K., Giosan, L., Richey, J. N., Nilsson-Kerr, K., Rosenthal, Y., Anand, P., and McGrath, S. M.: Remote and local drivers of Pleistocene South Asian summer monsoon precipitation: A test for future predictions, Sci. Adv., 7, eabg3848, https://doi.org/10.1126/sciadv.abg3848, 2021. a
Clement, A. C. and Peterson, L. C.: Mechanisms of abrupt climate change of the last glacial period, Rev. Geophys., 46, 1–39, https://doi.org/10.1029/2006RG000204, 2008. a, b
Cobb, K. M., Westphal, N., Sayani, H. R., Watson, J. T., Di Lorenzo, E., Cheng, H., Edwards, R., and Charles, C. D.: Highly variable El Niño–southern oscillation throughout the Holocene, Science, 339, 67–70, 2013. a
Coxall, H. K., Wilson, P. A., Pälike, H., Lear, C. H., and Backman, J.: Rapid stepwise onset of Antarctic glaciation and deeper calcite compensation in the Pacific Ocean, Nature, 433, 53–57, 2005. a, b, c
Coxall, H. K., Huck, C. E., Huber, M., Lear, C. H., Legarda-Lisarri, A., O’regan, M., Sliwinska, K. K., Van De Flierdt, T., De Boer, A. M., Zachos, J. C., and Backman, J.: Export of nutrient rich Northern Component Water preceded early Oligocene Antarctic glaciation, Nat. Geosci., 11, 190–196, 2018. a
Crawford, A., Stroeve, J., Smith, A., and Jahn, A.: Arctic open-water periods are projected to lengthen dramatically by 2100, Commun. Earth Environ., 2, 109, https://doi.org/10.1038/s43247-021-00183-x, 2021. a
Dansgaard, W., Johnsen, S. J., Clausen, H. B., Dahl-Jensen, D., Gundestrup, N. S., Hammer, C. U., Hvidberg, C. S., Steffensen, J. P., Sveinbjörnsdottir, A., Jouzel, J., and Bond, G.: Evidence for general instability of past climate from a 250-kyr ice-core record, Nature, 364, 218–220, 1993. a
Daron, J. D. and Stainforth, D. A.: On predicting climate under climate change, Environ. Res. Lett., 8, 034021, https://doi.org/10.1088/1748-9326/8/3/034021, 2013. a
De Noblet, N. I., Prentice, I. C., Joussaume, S., Texier, D., Botta, A., and Haxeltine, A.: Possible role of atmosphere-biosphere interactions in triggering the Last Glaciation, Geophys. Res. Lett., 23, 3191–3194, 1996. a
de Vrese, P., Georgievski, G., Gonzalez Rouco, J. F., Notz, D., Stacke, T., Steinert, N. J., Wilkenskjeld, S., and Brovkin, V.: Representation of soil hydrology in permafrost regions may explain large part of inter-model spread in simulated Arctic and subarctic climate, The Cryosphere, 17, 2095–2118, https://doi.org/10.5194/tc-17-2095-2023, 2023. a, b, c, d
de Vries, D., Heritage, S., Borths, M. R., Sallam, H. M., and Seiffert, E. R.: Widespread loss of mammalian lineage and dietary diversity in the early Oligocene of Afro-Arabia, Commun. Biol., 4, 1172, https://doi.org/10.1038/s42003-021-02707-9, 2021. a
Deb, P., Orr, A., Bromwich, D. H., Nicolas, J. P., Turner, J., and Hosking, J. S.: Summer drivers of atmospheric variability affecting ice shelf thinning in the Amundsen Sea Embayment, West Antarctica, Geophys. Res. Lett., 45, 4124–4133, 2018. a
Defrance, D., Ramstein, G., Charbit, S., Vrac, M., Famien, A. M., Sultan, B., Swingedouw, D., Dumas, C., Gemenne, F., Alvarez-Solas, J., and Vanderlinden, J.-P.: Consequences of rapid ice sheet melting on the Sahelian population vulnerability, P. Natl. Acad. Sci. USA, 114, 6533–6538, 2017. a, b
Dekker, M. M., von der Heydt, A. S., and Dijkstra, H. A.: Cascading transitions in the climate system, Earth Syst. Dynam., 9, 1243–1260, https://doi.org/10.5194/esd-9-1243-2018, 2018. a, b, c, d, e, f, g
Delworth, T. L. and Mann, M. E.: Observed and simulated multidecadal variability in the Northern Hemisphere, Clim. Dynam., 16, 661–676, 2000. a
Delworth, T. L., Zeng, F., Vecchi, G. A., Yang, X., Zhang, L., and Zhang, R.: The North Atlantic Oscillation as a driver of rapid climate change in the Northern Hemisphere, Nat. Geosci., 9, 509–512, 2016. a, b
Di Capua, G., Kretschmer, M., Donner, R. V., van den Hurk, B., Vellore, R., Krishnan, R., and Coumou, D.: Tropical and mid-latitude teleconnections interacting with the Indian summer monsoon rainfall: a theory-guided causal effect network approach, Earth Syst. Dynam., 11, 17–34, https://doi.org/10.5194/esd-11-17-2020, 2020. a
Dinniman, M. S., Asay-Davis, X. S., Galton-Fenzi, B. K., Holland, P. R., Jenkins, A., and Timmermann, R.: Modeling ice shelf/ocean interaction in Antarctica: A review, Oceanography, 29, 144–153, 2016. a
Docquier, D. and Koenigk, T.: A review of interactions between ocean heat transport and Arctic sea ice, Environ. Res. Lett., 16, 123002, https://doi.org/10.1088/1748-9326/ac30be, 2021. a
Docquier, D., Vannitsem, S., Ragone, F., Wyser, K., and Liang, X.: Causal links between Arctic sea ice and its potential drivers based on the rate of information transfer, Geophys. Res. Lett., 49, e2021GL095892, https://doi.org/10.1029/2021GL095892, 2022. a
Dommenget, D. and Latif, M.: Generation of hyper climate modes, Geophys. Res. Lett., 35, 2008. a, b
Donat-Magnin, M., Jourdain, N. C., Gallée, H., Amory, C., Kittel, C., Fettweis, X., Wille, J. D., Favier, V., Drira, A., and Agosta, C.: Interannual variability of summer surface mass balance and surface melting in the Amundsen sector, West Antarctica, The Cryosphere, 14, 229–249, https://doi.org/10.5194/tc-14-229-2020, 2020. a
Drüke, M., von Bloh, W., Petri, S., Sakschewski, B., Schaphoff, S., Forkel, M., Huiskamp, W., Feulner, G., and Thonicke, K.: CM2Mc-LPJmL v1.0: biophysical coupling of a process-based dynamic vegetation model with managed land to a general circulation model, Geosci. Model Dev., 14, 4117–4141, https://doi.org/10.5194/gmd-14-4117-2021, 2021. a
Duque-Villegas, M., Salazar, J. F., and Rendón, A. M.: Tipping the ENSO into a permanent El Niño can trigger state transitions in global terrestrial ecosystems, Earth Syst. Dynam., 10, 631–650, https://doi.org/10.5194/esd-10-631-2019, 2019. a, b
Dutton, A., Carlson, A. E., Long, A. J., Milne, G. A., Clark, P. U., DeConto, R., Horton, B. P., Rahmstorf, S., and Raymo, M. E.: Sea-level rise due to polar ice-sheet mass loss during past warm periods, Science, 349, 6244, https://doi.org/10.1126/science.aaa4019, 2015. a
D’Onofrio, D., Baudena, M., Lasslop, G., Nieradzik, L. P., Wårlind, D., and von Hardenberg, J.: Linking vegetation-climate-fire relationships in sub-Saharan Africa to key ecological processes in two dynamic global vegetation models, Frontiers in Environmental Science, 8, 136, https://doi.org/10.3389/fenvs.2020.00136, 2020. a
Eyring, V., Bony, S., Meehl, G. A., Senior, C. A., Stevens, B., Stouffer, R. J., and Taylor, K. E.: Overview of the Coupled Model Intercomparison Project Phase 6 (CMIP6) experimental design and organization, Geosci. Model Dev., 9, 1937–1958, https://doi.org/10.5194/gmd-9-1937-2016, 2016. a
Favier, L., Durand, G., Cornford, S. L., Gudmundsson, G. H., Gagliardini, O., Gillet-Chaulet, F., Zwinger, T., Payne, A., and Le Brocq, A. M.: Retreat of Pine Island Glacier controlled by marine ice-sheet instability, Nat. Clim. Change, 4, 117–121, 2014. a, b
Ferreira, D., Marshall, J., and Rose, B.: Climate determinism revisited: Multiple equilibria in a complex climate model, J. Clim., 24, 992–1012, 2011. a, b
Fisher, R. A., Koven, C. D., Anderegg, W. R., Christoffersen, B. O., Dietze, M. C., Farrior, C. E., Holm, J. A., Hurtt, G. C., Knox, R. G., Lawrence, P. J., Lichstein, J. W., Longo, M., Matheny, A. M., Medvigy, D., Muller-Landau, H. C., Powell, T. L., Serbin, S. P., Sato, H., Shuman, J. K., Smith, B., Trugman, A. T., Viskari, T., Verbeeck, H., Weng, E., Xu, C., Xu, X., Zhang, T., and Moorcroft, P. R.: Vegetation demographics in Earth System Models: A review of progress and priorities, Glob. Change Biol., 24, 35–54, 2018. a
Fletcher, W. J., Sánchez Goñi, M. F., Allen, J. R., Cheddadi, R., Combourieu-Nebout, N., Huntley, B., Lawson, I., Londeix, L., Magri, D., Margari, V., Müller, U. C., Naughton, F., Novenko, E., Roucoux, K., and Tzedakis, P.: Millennial-scale variability during the last glacial in vegetation records from Europe, Quaternary Sci. Rev., 29, 2839–2864, https://doi.org/10.1016/j.quascirev.2009.11.015, 2010. a
Foley, J. A., Levis, S., Prentice, I. C., Pollard, D., and Thompson, S. L.: Coupling dynamic models of climate and vegetation, Glob. Change Biol., 4, 561–579, 1998. a
Franzke, C. L., Ciullo, A., Gilmore, E. A., Matias, D. M., Nagabhatla, N., Orlov, A., Paterson, S. K., Scheffran, J., and Sillmann, J.: Perspectives on tipping points in integrated models of the natural and human Earth system: cascading effects and telecoupling, Environ. Res. Lett., 17, 015004, https://doi.org/10.1088/1748-9326/ac42fd, 2022. a
Frieler, K., Meinshausen, M., Golly, A., Mengel, M., Lebek, K., Donner, S., and Hoegh-Guldberg, O.: Limiting global warming to 2 ∘C is unlikely to save most coral reefs, Nat. Clim. Change, 3, 165–170, 2013. a
Fyke, J., Sergienko, O., Löfverström, M., Price, S., and Lenaerts, J. T.: An overview of interactions and feedbacks between ice sheets and the Earth system, Rev. Geophys., 56, 361–408, 2018. a, b
Ganopolski, A. and Rahmstorf, S.: Rapid changes of glacial climate simulated in a coupled climate model, Nature, 409, 153–158, 2001. a
Gatti, L. V., Basso, L. S., Miller, J. B., Gloor, M., Gatti Domingues, L., Cassol, H. L., Tejada, G., Aragão, L. E., Nobre, C., Peters, W., Marani, L., Arai, E., Sanches, A. H., Corrêa, S. M., Anderson, L., Von Randow, C., Correia, C. S., Crispim, S. P., and Neves, R. A.: Amazonia as a carbon source linked to deforestation and climate change, Nature, 595, 388–393, 2021. a
Gibson, K. A. and Peterson, L. C.: A 0.6 million year record of millennial-scale climate variability in the tropics, Geophys. Res. Lett., 41, 969–975, 2014. a
Gildor, H. and Tziperman, E.: Sea-ice switches and abrupt climate change, Philosophical Transactions of the Royal Society of London. Series A: Mathematical, Phys. Eng. Sci., 361, 1935–1944, 2003. a, b
Gomez, N., Weber, M. E., Clark, P. U., Mitrovica, J. X., and Han, H. K.: Antarctic ice dynamics amplified by Northern Hemisphere sea-level forcing, Nature, 587, 600–604, 2020. a, b, c
Granger, C. W.: Investigating causal relations by econometric models and cross-spectral methods, Econometrica: journal of the Econometric Society, 424–438, https://doi.org/10.2307/1912791, 1969. a
Häggi, C., Chiessi, C. M., Merkel, U., Mulitza, S., Prange, M., Schulz, M., and Schefuß, E.: Response of the Amazon rainforest to late Pleistocene climate variability, Earth Planet. Sc. Lett., 479, 50–59, 2017. a, b
Hankel, C. and Tziperman, E.: An approach for projecting the timing of abrupt winter Arctic sea ice loss, Nonlinear Proc. Geoph., 30, 299–309, 2023. a, b
Hawkins, E., Smith, R. S., Allison, L. C., Gregory, J. M., Woollings, T. J., Pohlmann, H., and De Cuevas, B.: Bistability of the Atlantic overturning circulation in a global climate model and links to ocean freshwater transport, Geophys. Res. Lett., 38, L10605, https://doi.org/10.1029/2011GL047208, 2011. a
Henry, L., McManus, J., Curry, W., Roberts, N., Piotrowski, A., and Keigwin, L.: North Atlantic ocean circulation and abrupt climate change during the last glaciation, Science, 353, 470–474, 2016. a, b
Herrington, A. R. and Poulsen, C. J.: Terminating the Last Interglacial: The role of ice sheet–climate feedbacks in a GCM asynchronously coupled to an ice sheet model, J. Clim., 25, 1871–1882, 2011. a
Hewitt, H., Fox-Kemper, B., Pearson, B., Roberts, M., and Klocke, D.: The small scales of the ocean may hold the key to surprises, Nat. Clim. Change, 12, 496–499, 2022. a
Hodell, D. A., Crowhurst, S. J., Lourens, L., Margari, V., Nicolson, J., Rolfe, J. E., Skinner, L. C., Thomas, N. C., Tzedakis, P. C., Mleneck-Vautravers, M. J., and Wolff, E. W.: A 1.5-million-year record of orbital and millennial climate variability in the North Atlantic, Clim. Past, 19, 607–636, https://doi.org/10.5194/cp-19-607-2023, 2023. a
Hooker, J. J., Collinson, M. E., and Sille, N. P.: Eocene–Oligocene mammalian faunal turnover in the Hampshire Basin, UK: calibration to the global time scale and the major cooling event, J. Geol. Soc., 161, 161–172, 2004. a
Hošeková, L., Eidam, E., Panteleev, G., Rainville, L., Rogers, W. E., and Thomson, J.: Landfast ice and coastal wave exposure in northern Alaska, Geophys. Res. Lett., 48, e2021GL095103, https://doi.org/10.1029/2021GL095103, 2021. a, b
Houk, P., Yalon, A., Maxin, S., Starsinic, C., McInnis, A., Gouezo, M., Golbuu, Y., and Van Woesik, R.: Predicting coral-reef futures from El Niño and Pacific Decadal Oscillation events, Sci. Rep., 10, 7735, https://doi.org/10.1038/s41598-020-64411-8, 2020. a
Hu, A., Meehl, G. A., Han, W., Lu, J., and Strand, W. G.: Energy balance in a warm world without the ocean conveyor belt and sea ice, Geophys. Res. Lett., 40, 6242–6246, 2013. a
Hughes, T. P., Carpenter, S., Rockström, J., Scheffer, M., and Walker, B.: Multiscale regime shifts and planetary boundaries, Trend. Ecol. Evol., 28, 389–395, 2013. a
Hughes, T. P., Anderson, K. D., Connolly, S. R., Heron, S. F., Kerry, J. T., Lough, J. M., Baird, A. H., Baum, J. K., Berumen, M. L., Bridge, T. C., Claar, D. C., Eakin, C. M., Gilmour, J. P., Graham, N. A. J., Harrison, H., Hobbs, J.-P. A., Hoey, A. S., Hoogenboom, M., Lowe, R. J., McCulloch, M. T., Pandolfi, J. M., Pratchett, M., Schoepf, V., Torda, G., and Wilson, S. K.: Spatial and temporal patterns of mass bleaching of corals in the Anthropocene, Science, 359, 80–83, 2018. a, b
Hutchinson, D. K., Coxall, H. K., Lunt, D. J., Steinthorsdottir, M., de Boer, A. M., Baatsen, M., von der Heydt, A., Huber, M., Kennedy-Asser, A. T., Kunzmann, L., Ladant, J.-B., Lear, C. H., Moraweck, K., Pearson, P. N., Piga, E., Pound, M. J., Salzmann, U., Scher, H. D., Sijp, W. P., Śliwińska, K. K., Wilson, P. A., and Zhang, Z.: The Eocene–Oligocene transition: a review of marine and terrestrial proxy data, models and model–data comparisons, Clim. Past, 17, 269–315, https://doi.org/10.5194/cp-17-269-2021, 2021. a, b
IPCC: IPCC Special Report on the Ocean and Cryosphere in a Changing Climate, edited by: Pörtner, H.-O., Roberts, D. C., Masson-Delmotte, V., Zhai, P., Tignor, M., Poloczanska, E., and Weyer, N., Cambridge University Press, Cambridge, UK and New York, NY, USA, 755 pp., https://doi.org/10.1017/9781009157964, 2019. a, b
Jaccard, S. L. and Galbraith, E. D.: Large climate-driven changes of oceanic oxygen concentrations during the last deglaciation, Nat. Geosci., 5, 151–156, 2012. a
Jackson, L., Kahana, R., Graham, T., Ringer, M., Woollings, T., Mecking, J., and Wood, R.: Global and European climate impacts of a slowdown of the AMOC in a high resolution GCM, Clim. Dynam., 45, 3299–3316, 2015. a, b, c, d, e
Jackson, L. C. and Wood, R. A.: Timescales of AMOC decline in response to fresh water forcing, Clim. Dynam., 51, 1333–1350, 2018. a, b
Jackson, R., Gabric, A., and Cropp, R.: Effects of ocean warming and coral bleaching on aerosol emissions in the Great Barrier Reef, Australia, Sci. Rep., 8, 14048, https://doi.org/10.1038/s41598-018-32470-7, 2018. a
Jansen, E., Christensen, J. H., Dokken, T., Nisancioglu, K. H., Vinther, B. M., Capron, E., Guo, C., Jensen, M. F., Langen, P. L., Pedersen, R. A., Yang, S., Bentsen, M., Kjær, H. A., Sadatzki, H., Sessford, E., and Stendel, M.: Past perspectives on the present era of abrupt Arctic climate change, Nat. Clim. Change, 10, 714–721, 2020. a
Jehn, F. U., Schneider, M., Wang, J. R., Kemp, L., and Breuer, L.: Betting on the best case: Higher end warming is underrepresented in research, Environ. Res. Lett., 16, 084036, https://doi.org/10.1088/1748-9326/ac13ef, 2021. a
Jiménez-Muñoz, J. C., Mattar, C., Barichivich, J., Santamaría-Artigas, A., Takahashi, K., Malhi, Y., Sobrino, J. A., and van der Schrier, G.: Record-breaking warming and extreme drought in the Amazon rainforest during the course of El Niño 2015–2016, Sci. Rep., 6, 33130, https://doi.org/10.1038/srep33130, 2016. a, b
Joughin, I., Smith, B. E., and Medley, B.: Marine ice sheet collapse potentially under way for the Thwaites Glacier Basin, West Antarctica, Science, 344, 735–738, 2014. a, b
Kanner, L. C., Burns, S. J., Cheng, H., and Edwards, R. L.: High-latitude forcing of the South American summer monsoon during the last glacial, Science, 335, 570–573, 2012. a
Kemp, L., Xu, C., Depledge, J., Ebi, K. L., Gibbins, G., Kohler, T. A., Rockström, J., Scheffer, M., Schellnhuber, H. J., Steffen, W., and Lenton, T.: Climate Endgame: Exploring catastrophic climate change scenarios, P. Natl. Acad. Sci. USA, 119, e2108146119, https://doi.org/10.1073/pnas.2108146119, 2022. a, b
Kennedy, A. T., Farnsworth, A., Lunt, D., Lear, C. H., and Markwick, P.: Atmospheric and oceanic impacts of Antarctic glaciation across the Eocene–Oligocene transition, Philos. T. R. Soc. A, 373, 20140419, https://doi.org/10.1098/rsta.2014.0419, 2015. a
Kim, H.-J., An, S.-I., Park, J.-H., Sung, M.-K., Kim, D., Choi, Y., and Kim, J.-S.: North Atlantic Oscillation impact on the Atlantic Meridional Overturning Circulation shaped by the mean state, npj Clim. Atmos. Sci., 6, 25, https://doi.org/10.1038/s41612-023-00354-x, 2023. a, b
Kleinen, T., Gromov, S., Steil, B., and Brovkin, V.: Atmospheric methane since the last glacial maximum was driven by wetland sources, Clim. Past, 19, 1081–1099, https://doi.org/10.5194/cp-19-1081-2023, 2023. a
Klose, A. K., Karle, V., Winkelmann, R., and Donges, J. F.: Emergence of cascading dynamics in interacting tipping elements of ecology and climate, Roy. Soc. Open Sci., 7, 200599, https://doi.org/10.1098/rsos.200599, 2020. a, b
Klose, A. K., Wunderling, N., Winkelmann, R., and Donges, J. F.: What do we mean,“tipping cascade”?, Environ. Res. Lett., 16, 125011, https://doi.org/10.1088/1748-9326/ac3955, 2021. a
Knight, J. R., Allan, R. J., Folland, C. K., Vellinga, M., and Mann, M. E.: A signature of persistent natural thermohaline circulation cycles in observed climate, Geophys. Res. Lett., 32, L20708, https://doi.org/10.1029/2005GL024233, 2005. a
Knorr, G. and Lohmann, G.: Rapid transitions in the Atlantic thermohaline circulation triggered by global warming and meltwater during the last deglaciation, Geochem. Geophy. Geosy., 8, 1–22, https://doi.org/10.1029/2007GC001604, 2007. a
Köhler, P., Knorr, G., and Bard, E.: Permafrost thawing as a possible source of abrupt carbon release at the onset of the Bølling/Allerød, Nat. Commun., 5, 5520, https://doi.org/10.1038/ncomms6520, 2014. a
Kopp, R. E., Mitrovica, J. X., Griffies, S. M., Yin, J., Hay, C. C., and Stouffer, R. J.: The impact of Greenland melt on local sea levels: a partially coupled analysis of dynamic and static equilibrium effects in idealized water-hosing experiments: a letter, Climatic Change, 103, 619–625, 2010. a, b
Korasidis, V. A., Wallace, M. W., Wagstaff, B. E., and Hill, R. S.: Terrestrial cooling record through the Eocene-Oligocene transition of Australia, Glob. Planet. Change, 173, 61–72, 2019. a
Kravtsov, S., Grimm, C., and Gu, S.: Global-scale multidecadal variability missing in state-of-the-art climate models, npj Clim. Atmos. Sci., 1, 34, https://doi.org/10.1038/s41612-018-0044-6, 2018. a, b
Krawczyk, H., Zinke, J., Browne, N., Struck, U., McIlwain, J., O’Leary, M., and Garbe-Schönberg, D.: Corals reveal ENSO-driven synchrony of climate impacts on both terrestrial and marine ecosystems in northern Borneo, Sci. Rep., 10, 3678, https://doi.org/10.1038/s41598-020-60525-1, 2020. a, b
Kretschmer, M., Coumou, D., Donges, J. F., and Runge, J.: Using causal effect networks to analyze different Arctic drivers of midlatitude winter circulation, J. Clim., 29, 4069–4081, 2016. a
Kretschmer, M., Adams, S. V., Arribas, A., Prudden, R., Robinson, N., Saggioro, E., and Shepherd, T. G.: Quantifying causal pathways of teleconnections, Bull. Am. Meteorol. Soc., 102, E2247–E2263, https://doi.org/10.1175/BAMS-D-20-0117.1, 2021. a
Kreuzer, M., Reese, R., Huiskamp, W. N., Petri, S., Albrecht, T., Feulner, G., and Winkelmann, R.: Coupling framework (1.0) for the PISM (1.1.4) ice sheet model and the MOM5 (5.1.0) ocean model via the PICO ice shelf cavity model in an Antarctic domain, Geosci. Model Dev., 14, 3697–3714, https://doi.org/10.5194/gmd-14-3697-2021, 2021. a, b
Kriegler, E., Hall, J. W., Held, H., Dawson, R., and Schellnhuber, H. J.: Imprecise probability assessment of tipping points in the climate system, P. Natl. Acad. Sci. USA, 106, 5041–5046, 2009. a, b, c
Kug, J.-S., Oh, J.-H., An, S.-I., Yeh, S.-W., Min, S.-K., Son, S.-W., Kam, J., Ham, Y.-G., and Shin, J.: Hysteresis of the intertropical convergence zone to CO2 forcing, Nat. Clim. Change, 12, 47–53, 2022. a
Kuhlbrodt, T., Griesel, A., Montoya, M., Levermann, A., Hofmann, M., and Rahmstorf, S.: On the driving processes of the Atlantic meridional overturning circulation, Rev. Geophys., 45, 1–32, 2007. a
Kukla, T., Ahlström, A., Maezumi, S. Y., Chevalier, M., Lu, Z., Winnick, M. J., and Chamberlain, C. P.: The resilience of Amazon tree cover to past and present drying, Glob. Planet. Change, 202, 103520, https://doi.org/10.1016/j.gloplacha.2021.103520, 2021. a, b
Landais, A., Jouzel, J., Masson-Delmotte, V., and Caillon, N.: Large temperature variations over rapid climatic events in Greenland: a method based on air isotopic measurements, C. R. Geosci., 337, 947–956, 2005. a
Le Nohaïc, M., Ross, C. L., Cornwall, C. E., Comeau, S., Lowe, R., McCulloch, M. T., and Schoepf, V.: Marine heatwave causes unprecedented regional mass bleaching of thermally resistant corals in northwestern Australia, Sci. Rep., 7, 14999, https://doi.org/10.1038/s41598-017-14794-y, 2017. a
Lear, C. H., Bailey, T. R., Pearson, P. N., Coxall, H. K., and Rosenthal, Y.: Cooling and ice growth across the Eocene-Oligocene transition, Geology, 36, 251–254, 2008. a, b
Lee, J.-Y., Marotzke, J., Bala, G., Cao, L., Corti, S., Dunne, J. P., Engelbrecht, F., Fischer, E., Fyfe, J. C., Jones, C., Maycock, A., Mutemi, J., Ndiaye, O., Panickal, S., and Zho, T.: Future global climate: scenario-based projections and near-term information, 553–672, Cambridge University Press, https://doi.org/10.1017/9781009157896.006, 2021. a
Lenton, T. M., Held, H., Kriegler, E., Hall, J. W., Lucht, W., Rahmstorf, S., and Schellnhuber, H. J.: Tipping elements in the Earth's climate system, P. Natl. Acad. Sci. USA, 105, 1786–1793, 2008. a, b
Lenton, T. M., Rockström, J., Gaffney, O., Rahmstorf, S., Richardson, K., Steffen, W., and Schellnhuber, H. J.: Climate tipping points—too risky to bet against, Nature, 575, 592–595, 2019. a, b
Levermann, A., Bamber, J. L., Drijfhout, S., Ganopolski, A., Haeberli, W., Harris, N. R., Huss, M., Krüger, K., Lenton, T. M., Lindsay, R. W., Notz, D., Wadhams, P., and Weber, S.: Potential climatic transitions with profound impact on Europe: Review of the current state of six “tipping elements of the climate system”, Climatic Change, 110, 845–878, 2012. a
Li, C., Battisti, D. S., and Bitz, C. M.: Can North Atlantic sea ice anomalies account for Dansgaard–Oeschger climate signals?, J. Clim., 23, 5457–5475, 2010. a
Li, H., Fedorov, A., and Liu, W.: AMOC stability and diverging response to Arctic sea ice decline in two climate models, J. Clim., 34, 5443–5460, 2021. a, b
Li, Q., Marshall, J., Rye, C. D., Romanou, A., Rind, D., and Kelley, M.: Global Climate Impacts of Greenland and Antarctic Meltwater: A Comparative Study, J. Clim., 36, 3571–3590, 2023. a
Liang, X. S.: Normalized multivariate time series causality analysis and causal graph reconstruction, Entropy, 23, 679, https://doi.org/10.3390/e23060679, 2021. a
Liang, X. S. and Kleeman, R.: Information transfer between dynamical system components, Phys. Rev. Lett., 95, 244101, https://doi.org/10.1103/PhysRevLett.95.244101, 2005. a
Liljedahl, A. K., Boike, J., Daanen, R. P., Fedorov, A. N., Frost, G. V., Grosse, G., Hinzman, L. D., Iijma, Y., Jorgenson, J. C., Matveyeva, N., Necsoiu, M., Raynolds, M. K., Romanovsky, V. E., Schulla, J., Tape, K. D., Walker, D. A., Wilson, C. J., Yabuki, H., and Zona, D.: Pan-Arctic ice-wedge degradation in warming permafrost and its influence on tundra hydrology, Nat. Geosci., 9, 312–318, 2016. a
Liu, T., Chen, D., Yang, L., Meng, J., Wang, Z., Ludescher, J., Fan, J., Yang, S., Chen, D., Kurths, J., Chen, X., Havlin, S., and Schellnhuber, H. J.: Teleconnections among tipping elements in the Earth system, Nat. Clim. Change, 13, 67–74, 2023. a, b, c
Liu, W. and Fedorov, A.: Interaction between Arctic sea ice and the Atlantic meridional overturning circulation in a warming climate, Clim. Dynam., 58, 1811–1827, https://doi.org/10.1007/s00382-021-05993-5, 2022. a, b, c, d, e
Liu, W., Fedorov, A. V., Xie, S.-P., and Hu, S.: Climate impacts of a weakened Atlantic Meridional Overturning Circulation in a warming climate, Sci. Adv., 6, eaaz4876, https://doi.org/10.1126/sciadv.aaz4876, 2020. a, b, c
Liu, Z., Otto-Bliesner, B. L., He, F., Brady, E. C., Tomas, R., Clark, P. U., Carlson, A. E., Lynch-Stieglitz, J., Curry, W., Brook, E., Erickson, D., Jacob, R., Kutzbach, J., and Cheng, J.: Transient Simulation of Last Deglaciation with a New Mechanism for Bølling-Allerød Warming, Science, 325, 310–314, 2009. a
Lohmann, J.: Prediction of Dansgaard-Oeschger Events From Greenland Dust Records, Geophys. Res. Lett., 46, 12427–12434, 2019. a
Lohmann, J. and Ditlevsen, P. D.: Risk of tipping the overturning circulation due to increasing rates of ice melt, P. Natl. Acad. Sci. USA, 118, e2017989118, https://doi.org/10.1073/pnas.2017989118, 2021. a
Lohmann, J., Castellana, D., Ditlevsen, P. D., and Dijkstra, H. A.: Abrupt climate change as a rate-dependent cascading tipping point, Earth Syst. Dynam., 12, 819–835, https://doi.org/10.5194/esd-12-819-2021, 2021. a
Lough, J., Anderson, K., and Hughes, T.: Increasing thermal stress for tropical coral reefs: 1871–2017, Sci. Rep., 8, 6079, https://doi.org/10.1038/s41598-018-24530-9, 2018. a, b, c
Lovejoy, T. E. and Nobre, C.: Amazon tipping point, Sci. Adv., 4, eaat2340, https://doi.org/10.1126/sciadv.aat2340, 2018. a
Lucarini, V. and Bódai, T.: Edge states in the climate system: exploring global instabilities and critical transitions, Nonlinearity, 30, R32–R66, https://doi.org/10.1088/1361-6544/aa6b11, 2017. a
MacAyeal, D. R.: Binge/purge oscillations of the Laurentide ice sheet as a cause of the North Atlantic's Heinrich events, Paleoceanography, 8, 775–784, 1993. a
Madsen, M., Yang, S., Aðalgeirsdóttir, G., Svendsen, S., Rodehacke, C., and Ringgaard, I.: The role of an interactive Greenland ice sheet in the coupled climate-ice sheet model EC-Earth-PISM, Clim. Dynam., 59, 1189–1211, 2022. a, b
Mahendra, N., Chowdary, J. S., Darshana, P., Sunitha, P., Parekh, A., and Gnanaseelan, C.: Interdecadal modulation of interannual ENSO-Indian summer monsoon rainfall teleconnections in observations and CMIP6 models: Regional patterns, Int. J. Climatol., 41, 2528–2552, 2021. a
Makarieva, A. M., Nefiodov, A. V., Nobre, A. D., Baudena, M., Bardi, U., Sheil, D., Saleska, S. R., Molina, R. D., and Rammig, A.: The role of ecosystem transpiration in creating alternate moisture regimes by influencing atmospheric moisture convergence, Glob. Change Biol., 29, 2536–2556, 2023. a
Manabe, S. and Stouffer, R. J.: Simulation of abrupt climate change induced by freshwater input to the North Atlantic Ocean, Nature, 378, 165–167, 1995. a
Marcott, S. A., Bauska, T. K., Buizert, C., Steig, E. J., Rosen, J. L., Cuffey, K. M., Fudge, T., Severinghaus, J. P., Ahn, J., Kalk, M. L., McConnell, J. R., Sowers, T., Taylor, K. C., White, J. W., and Brook, E. J.: Centennial-scale changes in the global carbon cycle during the last deglaciation, Nature, 514, 616–619, 2014. a
Martin, T. and Biastoch, A.: On the ocean's response to enhanced Greenland runoff in model experiments: relevance of mesoscale dynamics and atmospheric coupling, Ocean Sci., 19, 141–167, https://doi.org/10.5194/os-19-141-2023, 2023. a
Martrat, B., Grimalt, J. O., Shackleton, N. J., de Abreu, L., Hutterli, M. A., and Stocker, T. F.: Four climate cycles of recurring deep and surface water destabilizations on the Iberian margin, Science, 317, 502–507, 2007. a
Masson-Delmotte, V. P., Zhai, A., Pirani, S., Connors, C., Péan, S., Berger, N., Caud, Y., Chen, L., Goldfarb, M., Gomis, M., Huang, K., Leitzell, E., Lonnoy, J., Matthews, T., Maycock, T., Waterfield, O., Yelekci, R. Y., and Zhou, B. E.: IPCC, 2021: Climate Change 2021: The Physical Science Basis, Contribution of Working Group I to the Sixth Assessment Report of the Intergovernmental Panel on Climate Change, Cambridge University Press, Cambridge, United Kingdom and New York, NY, USA, https://doi.org/10.1017/9781009157896, 2021. a, b, c, d
Mastrandrea, M. D., Mach, K. J., Plattner, G.-K., Edenhofer, O., Stocker, T. F., Field, C. B., Ebi, K. L., and Matschoss, P. R.: The IPCC AR5 guidance note on consistent treatment of uncertainties: a common approach across the working groups, Climatic Change, 108, 675–691, 2011. a
Max, L., Nürnberg, D., Chiessi, C. M., Lenz, M. M., and Mulitza, S.: Subsurface ocean warming preceded Heinrich Events, Nat. Commun., 13, 4217, https://doi.org/10.1038/s41467-022-31754-x, 2022. a
McGowan, H. and Theobald, A.: Atypical weather patterns cause coral bleaching on the Great Barrier Reef, Australia during the 2021–2022 La Niña, Sci. Rep., 13, 6397, https://doi.org/10.1038/s41598-023-33613-1, 2023. a
McManus, J. F., Francois, R., Gherardi, J.-M., Keigwin, L. D., and Brown-Leger, S.: Collapse and rapid resumption of Atlantic meridional circulation linked to deglacial climate changes, Nature, 428, 834–837, 2004. a
McPhaden, M. J., Zebiak, S. E., and Glantz, M. H.: ENSO as an integrating concept in earth science, Science, 314, 1740–1745, 2006. a
Mecking, J., Drijfhout, S. S., Jackson, L. C., and Graham, T.: Stable AMOC off state in an eddy-permitting coupled climate model, Clim. Dynam., 47, 2455–2470, 2016. a, b, c
Mitrovica, J. X., Gomez, N., and Clark, P. U.: The sea-level fingerprint of West Antarctic collapse, Science, 323, 753–753, 2009. a, b
Muñiz-Castillo, A. I., Rivera-Sosa, A., Chollett, I., Eakin, C. M., Andrade-Gómez, L., McField, M., and Arias-González, J. E.: Three decades of heat stress exposure in Caribbean coral reefs: a new regional delineation to enhance conservation, Sci. Rep., 9, 11013, https://doi.org/10.1038/s41598-019-47307-0, 2019. a, b, c
Muntjewerf, L., Petrini, M., Vizcaino, M., Ernani da Silva, C., Sellevold, R., Scherrenberg, M. D., Thayer-Calder, K., Bradley, S. L., Lenaerts, J. T., Lipscomb, W. H., and Lofverstrom, M.: Greenland Ice Sheet contribution to 21st century sea level rise as simulated by the coupled CESM2, 1-CISM2, 1, Geophys. Res. Lett., 47, e2019GL086836, https://doi.org/10.1029/2019GL086836, 2020. a, b
Murphy, J. M., Sexton, D. M., Barnett, D. N., Jones, G. S., Webb, M. J., Collins, M., and Stainforth, D. A.: Quantification of modelling uncertainties in a large ensemble of climate change simulations, Nature, 430, 768–772, 2004. a, b
NEEM community members: Eemian interglacial reconstructed from a Greenland folded ice core, Nature, 493, 489–494, 2013. a
Nian, D., Bathiany, S., Ben-Yami, M., Blaschke, L. L., Hirota, M., Rodrigues, R. R., and Boers, N.: A potential collapse of the Atlantic Meridional Overturning Circulation may stabilise eastern Amazonian rainforests, Communications Earth and Environment, 4, p. 470, https://doi.org/10.1038/s43247-023-01123-7, 2023. a, b
Nicolas, J. P., Vogelmann, A. M., Scott, R. C., Wilson, A. B., Cadeddu, M. P., Bromwich, D. H., Verlinde, J., Lubin, D., Russell, L. M., Jenkinson, C., Powers, H. H., Ryczek, M., Stone, G., and Wille, J. D.: January 2016 extensive summer melt in West Antarctica favoured by strong El Niño, Nat. Commun., 8, 15799, https://doi.org/10.1038/ncomms15799, 2017. a, b
Niederdrenk, A. L. and Notz, D.: Arctic sea ice in a 1.5 ∘C warmer world, Geophys. Res. Lett., 45, 1963–1971, 2018. a
Nielsen, D. M., Dobrynin, M., Baehr, J., Razumov, S., and Grigoriev, M.: Coastal erosion variability at the southern Laptev Sea linked to winter sea ice and the Arctic Oscillation, Geophys. Res. Lett., 47, e2019GL086876, https://doi.org/10.1029/2019GL086876, 2020. a
Nielsen, D. M., Pieper, P., Barkhordarian, A., Overduin, P., Ilyina, T., Brovkin, V., Baehr, J., and Dobrynin, M.: Increase in Arctic coastal erosion and its sensitivity to warming in the twenty-first century, Nat. Clim. Change, 12, 263–270, 2022. a, b
Nilsson-Kerr, K., Anand, P., Sexton, P., Leng, M., Misra, S., Clemens, S., and Hammond, S.: Role of Asian summer monsoon subsystems in the inter-hemispheric progression of deglaciation, Nat. Geosci., 12, 290–295, 2019. a
Nilsson-Kerr, K., Anand, P., Sexton, P. F., Leng, M. J., and Naidu, P. D.: Indian Summer Monsoon variability 140–70 thousand years ago based on multi-proxy records from the Bay of Bengal, Quaternary Sci. Rev., 279, 107403, https://doi.org/10.1016/j.quascirev.2022.107403, 2022. a
Nitzbon, J., Westermann, S., Langer, M., Martin, L. C., Strauss, J., Laboor, S., and Boike, J.: Fast response of cold ice-rich permafrost in northeast Siberia to a warming climate, Nat. Commun., 11, 2201, https://doi.org/10.1038/s41467-020-15725-8, 2020. a, b
Nobre, C. A., Sampaio, G., Borma, L. S., Castilla-Rubio, J. C., Silva, J. S., and Cardoso, M.: Land-use and climate change risks in the Amazon and the need of a novel sustainable development paradigm, P. Natl. Acad. Sci. USA, 113, 10759–10768, 2016. a
Noël, B., van de Berg, W. J., van Wessem, J. M., van Meijgaard, E., van As, D., Lenaerts, J. T. M., Lhermitte, S., Kuipers Munneke, P., Smeets, C. J. P. P., van Ulft, L. H., van de Wal, R. S. W., and van den Broeke, M. R.: Modelling the climate and surface mass balance of polar ice sheets using RACMO2 – Part 1: Greenland (1958–2016), The Cryosphere, 12, 811–831, https://doi.org/10.5194/tc-12-811-2018, 2018. a
North Greenland Ice Core Project members (NGRIP): High-resolution record of Northern Hemisphere climate extending into the last interglacial period, Nature, 431, 147–151, 2004. a
Notz, D. and Stroeve, J.: Observed Arctic sea-ice loss directly follows anthropogenic CO2 emission, Science, 354, 747–750, 2016. a
Novello, V. F., Cruz, F. W., Vuille, M., Stríkis, N. M., Edwards, R. L., Cheng, H., Emerick, S., De Paula, M. S., Li, X., Barreto, E. D. S., Karmann, I., and Santos, R. V.: A high-resolution history of the South American Monsoon from Last Glacial Maximum to the Holocene, Sci. Rep., 7, 44267, https://doi.org/10.1038/srep44267, 2017. a
Obura, D. O., Bigot, L., and Benzoni, F.: Coral responses to a repeat bleaching event in Mayotte in 2010, PeerJ, 6, e5305, https://doi.org/10.7717/peerj.5305, 2018. a
Onarheim, I. H., Eldevik, T., Årthun, M., Ingvaldsen, R. B., and Smedsrud, L. H.: Skillful prediction of Barents Sea ice cover, Geophys. Res. Lett., 42, 5364–5371, 2015. a
Orihuela-Pinto, B., England, M. H., and Taschetto, A. S.: Interbasin and interhemispheric impacts of a collapsed Atlantic Overturning Circulation, Nat. Clim. Change, 12, 558–565, 2022a. a, b, c, d, e
Orihuela-Pinto, B., Santoso, A., England, M. H., and Taschetto, A. S.: Reduced ENSO variability due to a collapsed Atlantic Meridional Overturning Circulation, J. Clim., 35, 5307–5320, 2022b. a, b
Palacio-Castro, A. M., Smith, T. B., Brandtneris, V., Snyder, G. A., van Hooidonk, R., Maté, J. L., Manzello, D., Glynn, P. W., Fong, P., and Baker, A. C.: Increased dominance of heat-tolerant symbionts creates resilient coral reefs in near-term ocean warming, P. Natl. Acad. Sci. USA, 120, e2202388120, https://doi.org/10.1073/pnas.2202388120, 2023. a
Pandey, P., Dwivedi, S., Goswami, B., and Kucharski, F.: A new perspective on ENSO-Indian summer monsoon rainfall relationship in a warming environment, Clim. Dynam., 55, 3307–3326, 2020. a
Paolo, F., Padman, L., Fricker, H., Adusumilli, S., Howard, S., and Siegfried, M.: Response of Pacific-sector Antarctic ice shelves to the El Niño/Southern oscillation, Nat. Geosci., 11, 121–126, 2018. a, b
Parsons, L. A., Yin, J., Overpeck, J. T., Stouffer, R. J., and Malyshev, S.: Influence of the Atlantic Meridional Overturning Circulation on the monsoon rainfall and carbon balance of the American tropics, Geophys. Res. Lett., 41, 146–151, 2014. a, b
Pearl, J.: Bayesian networks: A model of self-activated memory for evidential reasoning, in: Proceedings of the 7th conference of the Cognitive Science Society, University of California, Irvine, CA, USA, 1–14, 1985. a
Pedersen, R. A. and Christensen, J. H.: Attributing Greenland warming patterns to regional Arctic sea ice loss, Geophys. Res. Lett., 46, 10495–10503, 2019. a, b
Pedro, J. B., Jochum, M., Buizert, C., He, F., Barker, S., and Rasmussen, S. O.: Beyond the bipolar seesaw: Toward a process understanding of interhemispheric coupling, Quaternary Sci. Rev., 192, 27–46, 2018. a, b
Pohl, A., Donnadieu, Y., Le Hir, G., Ladant, J.-B., Dumas, C., Alvarez-Solas, J., and Vandenbroucke, T. R.: Glacial onset predated Late Ordovician climate cooling, Paleoceanography, 31, 800–821, 2016. a
Pollard, D.: A retrospective look at coupled ice sheet–climate modeling, Climatic Change, 100, 173–194, 2010. a
Polyakov, I. V., Pnyushkov, A. V., Alkire, M. B., Ashik, I. M., Baumann, T. M., Carmack, E. C., Goszczko, I., Guthrie, J., Ivanov, V. V., Kanzow, T., Krishfield, R., Kwok, R., Sundfjord, A., Morison, J., Rember, R., and Yulin, A.: Greater role for Atlantic inflows on sea-ice loss in the Eurasian Basin of the Arctic Ocean, Science, 356, 285–291, 2017. a
Popp, M., Schmidt, H., and Marotzke, J.: Transition to a moist greenhouse with CO2 and solar forcing, Nat. Commun., 7, 10627, https://doi.org/10.1038/ncomms10627, 2016. a
Pöppelmeier, F., Joos, F., and Stocker, T. F.: The Coupled Ice Sheet–Earth System Model Bern3D v3. 0, J. Clim., 36, 7563–7582, 2023. a
Prado, L. F., Wainer, I., Chiessi, C. M., Ledru, M.-P., and Turcq, B.: A mid-Holocene climate reconstruction for eastern South America, Clim. Past, 9, 2117–2133, https://doi.org/10.5194/cp-9-2117-2013, 2013. a
Praetorius, S. K., Mix, A. C., Walczak, M., Wolhowe, M. D., Addison, J. A., and Prahl, F. G.: North Pacific deglacial hypoxic events linked to abrupt ocean warming, Nature, 527, 362–366, 2015. a
Quiquet, A., Roche, D. M., Dumas, C., Bouttes, N., and Lhardy, F.: Climate and ice sheet evolutions from the last glacial maximum to the pre-industrial period with an ice-sheet–climate coupled model, Clim. Past, 17, 2179–2199, https://doi.org/10.5194/cp-17-2179-2021, 2021. a
Rae, J. G. L., Aðalgeirsdóttir, G., Edwards, T. L., Fettweis, X., Gregory, J. M., Hewitt, H. T., Lowe, J. A., Lucas-Picher, P., Mottram, R. H., Payne, A. J., Ridley, J. K., Shannon, S. R., van de Berg, W. J., van de Wal, R. S. W., and van den Broeke, M. R.: Greenland ice sheet surface mass balance: evaluating simulations and making projections with regional climate models, The Cryosphere, 6, 1275–1294, https://doi.org/10.5194/tc-6-1275-2012, 2012. a
Rahmstorf, S.: On the freshwater forcing and transport of the Atlantic thermohaline circulation, Clim. Dynam., 12, 799–811, 1996. a
Reichart, G.-J., Lourens, L., and Zachariasse, W.: Temporal variability in the northern Arabian Sea oxygen minimum zone (OMZ) during the last 225,000 years, Paleoceanography, 13, 607–621, 1998. a
Rietkerk, M., Bastiaansen, R., Banerjee, S., van de Koppel, J., Baudena, M., and Doelman, A.: Evasion of tipping in complex systems through spatial pattern formation, Science, 374, eabj0359, https://doi.org/10.1126/science.abj0359, 2021. a, b
Ritchie, P. D., Clarke, J. J., Cox, P. M., and Huntingford, C.: Overshooting tipping point thresholds in a changing climate, Nature, 592, 517–523, 2021. a
Rocha, J. C., Peterson, G., Bodin, Ö., and Levin, S.: Cascading regime shifts within and across scales, Science, 362, 1379–1383, 2018. a, b, c
Rosier, S. H. R., Reese, R., Donges, J. F., De Rydt, J., Gudmundsson, G. H., and Winkelmann, R.: The tipping points and early warning indicators for Pine Island Glacier, West Antarctica, The Cryosphere, 15, 1501–1516, https://doi.org/10.5194/tc-15-1501-2021, 2021. a
Runge, J., Petoukhov, V., Donges, J. F., Hlinka, J., Jajcay, N., Vejmelka, M., Hartman, D., Marwan, N., Paluš, M., and Kurths, J.: Identifying causal gateways and mediators in complex spatio-temporal systems, Nat. Commun., 6, 1–10, 2015. a
Runge, J., Bathiany, S., Bollt, E., Camps-Valls, G., Coumou, D., Deyle, E., Glymour, C., Kretschmer, M., Mahecha, M. D., Muñoz-Marí, J., van Nes, E., Peters, J., Quax, R., Reichstein, M., Scheffer, M., Schölkopf, B., Spirtes, P., Sugihara, G., Sun, J., Zhang, K., and Zscheischler, J.: Inferring causation from time series in Earth system sciences, Nat. Commun., 10, 2553, https://doi.org/10.1038/s41467-019-10105-3, 2019a. a
Runge, J., Nowack, P., Kretschmer, M., Flaxman, S., and Sejdinovic, D.: Detecting and quantifying causal associations in large nonlinear time series datasets, Sci. Adv., 5, eaau4996, https://doi.org/10.1126/sciadv.aau4996, 2019b. a
Ruth, U., Bigler, M., Röthlisberger, R., Siggaard-Andersen, M.-L., Kipfstuhl, S., Goto-Azuma, K., Hansson, M. E., Johnsen, S. J., Lu, H., and Steffensen, J. P.: Ice core evidence for a very tight link between North Atlantic and east Asian glacial climate, Geophys. Res. Lett., 34, L03706, https://doi.org/10.1029/2006GL027876, 2007. a
Sadai, S., Condron, A., DeConto, R., and Pollard, D.: Future climate response to Antarctic Ice Sheet melt caused by anthropogenic warming, Sci. Adv., 6, eaaz1169, https://doi.org/10.1126/sciadv.aaz1169, 2020. a
Sadatzki, H., Maffezzoli, N., Dokken, T. M., Simon, M. H., Berben, S. M. P., Fahl, K., Kjær, H. A., Spolaor, A., Stein, R., Vallelonga, P., Vinther, B. M., and Jansen, E.: Rapid reductions and millennial-scale variability in Nordic Seas sea ice cover during abrupt glacial climate changes, P. Natl. Acad. Sci. USA, 117, 29478–29486, 2020. a
Schannwell, C., Mikolajewicz, U., Ziemen, F., and Kapsch, M.-L.: Sensitivity of Heinrich-type ice-sheet surge characteristics to boundary forcing perturbations, Clim. Past, 19, 179–198, https://doi.org/10.5194/cp-19-179-2023, 2023. a, b
Scherrenberg, M. D. W., Berends, C. J., Stap, L. B., and van de Wal, R. S. W.: Modelling feedbacks between the Northern Hemisphere ice sheets and climate during the last glacial cycle, Clim. Past, 19, 399–418, https://doi.org/10.5194/cp-19-399-2023, 2023. a
Schleussner, C.-F., Lissner, T. K., Fischer, E. M., Wohland, J., Perrette, M., Golly, A., Rogelj, J., Childers, K., Schewe, J., Frieler, K., Mengel, M., Hare, W., and Schaeffer, M.: Differential climate impacts for policy-relevant limits to global warming: the case of 1.5 ∘C and 2 ∘C, Earth Syst. Dynam., 7, 327–351, https://doi.org/10.5194/esd-7-327-2016, 2016. a
Schneider, T., Bischoff, T., and Haug, G. H.: Migrations and dynamics of the intertropical convergence zone, Nature, 513, 45–53, 2014. a
Schneider, T., Kaul, C. M., and Pressel, K. G.: Possible climate transitions from breakup of stratocumulus decks under greenhouse warming, Nat. Geosci., 12, 163–167, 2019. a
Schoof, C.: Ice sheet grounding line dynamics: Steady states, stability, and hysteresis, J. Geophys. Res.-Earth, 112, 1–19, 2007. a
Schutz, B. E., Zwally, H. J., Shuman, C. A., Hancock, D., and DiMarzio, J. P.: Overview of the ICESat mission, Geophys. Res. Lett., 32, L21S01, https://doi.org/10.1029/2005GL024009, 2005. a
Schwinger, J., Asaadi, A., Steinert, N. J., and Lee, H.: Emit now, mitigate later? Earth system reversibility under overshoots of different magnitudes and durations, Earth Syst. Dynam., 13, 1641–1665, https://doi.org/10.5194/esd-13-1641-2022, 2022. a, b
Scott, R. C., Nicolas, J. P., Bromwich, D. H., Norris, J. R., and Lubin, D.: Meteorological drivers and large-scale climate forcing of West Antarctic surface melt, J. Clim., 32, 665–684, 2019. a, b, c, d
Seidov, D., Stouffer, R. J., and Haupt, B. J.: Is there a simple bi-polar ocean seesaw?, Glob. Planet. Change, 49, 19–27, 2005. a, b
Sévellec, F., Fedorov, A. V., and Liu, W.: Arctic sea-ice decline weakens the Atlantic meridional overturning circulation, Nat. Clim. Change, 7, 604–610, 2017. a, b, c, d
Severinghaus, J. P. and Brook, E. J.: Abrupt climate change at the end of the last glacial period inferred from trapped air in polar ice, Science, 286, 930–934, 1999. a
Shackleton, N. J., Hall, M. A., and Vincent, E.: Phase relationships between millennial-scale events 64,000–24,000 years ago, Paleoceanography, 15, 565–569, 2000. a
Shin, Y. and Kang, S. M.: How Does the High-Latitude Thermal Forcing in One Hemisphere Affect the Other Hemisphere?, Geophys. Res. Lett., 48, e2021GL095870, https://doi.org/10.1029/2021GL095870, 2021. a
Sinet, S., von der Heydt, A., and Dijkstra, H.: AMOC stabilization under the interaction with tipping polar ice sheets, Geophys. Res. Lett., 50, e2022GL100305, https://doi.org/10.1029/2022GL100305, 2023. a, b, c
Slingo, J., Bates, P., Bauer, P., Belcher, S., Palmer, T., Stephens, G., Stevens, B., Stocker, T., and Teutsch, G.: Ambitious partnership needed for reliable climate prediction, Nat. Clim. Change, 12, 499–503, 2022. a
Solomon, A., Heuzé, C., Rabe, B., Bacon, S., Bertino, L., Heimbach, P., Inoue, J., Iovino, D., Mottram, R., Zhang, X., Aksenov, Y., McAdam, R., Nguyen, A., Raj, R. P., and Tang, H.: Freshwater in the Arctic Ocean 2010–2019, Ocean Sci., 17, 1081–1102, https://doi.org/10.5194/os-17-1081-2021, 2021. a
Srivastava, G., Chakraborty, A., and Nanjundiah, R. S.: Multidecadal see-saw of the impact of ENSO on Indian and West African summer monsoon rainfall, Clim. Dynam., 52, 6633–6649, 2019. a
Srokosz, M. and Bryden, H.: Observing the Atlantic Meridional Overturning Circulation yields a decade of inevitable surprises, Science, 348, 1255575, https://doi.org/10.1126/science.1255575, 2015. a
Staal, A., Tuinenburg, O. A., Bosmans, J. H., Holmgren, M., van Nes, E. H., Scheffer, M., Zemp, D. C., and Dekker, S. C.: Forest-rainfall cascades buffer against drought across the Amazon, Nat. Clim. Change, 8, 539–543, 2018. a
Staal, A., Fetzer, I., Wang-Erlandsson, L., Bosmans, J. H., Dekker, S. C., van Nes, E. H., Rockström, J., and Tuinenburg, O. A.: Hysteresis of tropical forests in the 21st century, Nat. Commun., 11, 1–8, 2020. a, b
Stainforth, D. A., Downing, T. E., Washington, R., Lopez, A., and New, M.: Issues in the interpretation of climate model ensembles to inform decisions, Philos. T. R. Soc. A, 365, 2163–2177, 2007. a
Steffen, W.: Introducing the Anthropocene: The Human Epoch, Ambio, 50, 1784–1787, 2021. a
Steffen, W., Rockström, J., Richardson, K., Lenton, T. M., Folke, C., Liverman, D., Summerhayes, C. P., Barnosky, A. D., Cornell, S. E., Crucifix, M., Donges, J. F., Fetzer, I., Lade, S. J., Scheffer, M., Winkelmann, R., and Schellnhuber, H. J.: Trajectories of the Earth System in the Anthropocene, P. Natl. Acad. Sci. USA, 115, 8252–8259, 2018. a, b, c, d, e
Steffensen, J. P., Andersen, K. K., Bigler, M., Clausen, H. B., Dahl-Jensen, D., Fischer, H., Goto-Azuma, K., Hansson, M., Johnsen, S. J., Jouzel, J., Masson-Delmotte, V., Popp, T., Rasmussen, S. O., Röthlisberger, R., Ruth, U., Stauffer, B., Siggaard-Andersen, M.-L., Árný E. Sveinbjörnsdóttir, Svensson, A., and White, J. W. C.: High-Resolution Greenland Ice Core Data Show Abrupt Climate Change Happens in Few Years, Science, 321, 680–684, 2008. a
Stocker, T. F. and Johnsen, S. J.: A minimum thermodynamic model for the bipolar seesaw, Paleoceanography, 18, 1–9, https://doi.org/10.1029/2003PA000920, 2003. a, b, c
Stommel, H.: Thermohaline convection with two stable regimes of flow, Tellus, 13, 224–230, 1961. a, b
Stouffer, R. J., Yin, J.-j., Gregory, J., Dixon, K., Spelman, M., Hurlin, W., Weaver, A., Eby, M., Flato, G., Hasumi, H., Hu, A., Jungclaus, J., Kamenkovich, I., Levermann, A., Montoya, M., Murakami, S., Nawrath, S., Oka, A., Peltier, W., Robitaille, D., Sokolov, A., Vettoretti, G., and Weber, S.: Investigating the causes of the response of the thermohaline circulation to past and future climate changes, J. Clim., 19, 1365–1387, 2006. a, b, c, d
Stouffer, R. J., Seidov, D., and Haupt, B. J.: Climate response to external sources of freshwater: North Atlantic versus the Southern Ocean, J. Clim., 20, 436–448, 2007. a, b, c, d
Sun, Y., Clemens, S. C., Morrill, C., Lin, X., Wang, X., and An, Z.: Influence of Atlantic meridional overturning circulation on the East Asian winter monsoon, Nat. Geosci., 5, 46–49, 2012. a
Sutter, J., Jones, A., Frölicher, T., Wirths, C., and Stocker, T.: Climate intervention on a high-emissions pathway could delay but not prevent West Antarctic Ice Sheet demise, Nat. Clim. Change, 13, 951–960, 2023. a
Svendsen, L., Kvamstø, N. G., and Keenlyside, N.: Weakening AMOC connects equatorial Atlantic and Pacific interannual variability, Clim. Dynam., 43, 2931–2941, 2014. a
Swingedouw, D., Braconnot, P., and Marti, O.: Sensitivity of the Atlantic Meridional Overturning Circulation to the melting from northern glaciers in climate change experiments, Geophys. Res. Lett., 33, L07711, https://doi.org/10.1029/2006GL025765, 2006. a
Swingedouw, D., Braconnot, P., Delécluse, P., Guilyardi, E., and Marti, O.: Quantifying the AMOC feedbacks during a 2× CO2 stabilization experiment with land-ice melting, Clim. Dynam., 29, 521–534, 2007. a
Swingedouw, D., Fichefet, T., Huybrechts, P., Goosse, H., Driesschaert, E., and Loutre, M.-F.: Antarctic ice-sheet melting provides negative feedbacks on future climate warming, Geophys. Res. Lett., 35, L17705, https://doi.org/10.1029/2008GL034410, 2008. a, b, c
Swingedouw, D., Fichefet, T., Goosse, H., and Loutre, M.-F.: Impact of transient freshwater releases in the Southern Ocean on the AMOC and climate, Clim. Dynam., 33, 365–381, 2009. a, b, c, d
Swingedouw, D., Rodehacke, C. B., Behrens, E., Menary, M., Olsen, S. M., Gao, Y., Mikolajewicz, U., Mignot, J., and Biastoch, A.: Decadal fingerprints of freshwater discharge around Greenland in a multi-model ensemble, Clim. Dynam., 41, 695–720, 2013. a, b
Swingedouw, D., Houssais, M.-N., Herbaut, C., Blaizot, A.-C., Devilliers, M., and Deshayes, J.: AMOC recent and future trends: a crucial role for oceanic resolution and Greenland melting?, Front. Clim., 4, 838310, https://doi.org/10.3389/fclim.2022.838310, 2022. a
Thomas, Z. A., Jones, R. T., Turney, C. S., Golledge, N., Fogwill, C., Bradshaw, C. J., Menviel, L., McKay, N. P., Bird, M., Palmer, J., Kershaw, P., Wilmshurst, J., and Muscheler, R.: Tipping elements and amplified polar warming during the Last Interglacial, Quaternary Sci. Rev., 233, 106222, https://doi.org/10.1016/j.quascirev.2020.106222, 2020. a
Tietsche, S., Notz, D., Jungclaus, J., and Marotzke, J.: Recovery mechanisms of Arctic summer sea ice, Geophys. Res. Lett., 38, L02707, https://doi.org/10.1029/2010GL045698, 2011. a
Tigchelaar, M., von der Heydt, A. S., and Dijkstra, H. A.: A new mechanism for the two-step δ18O signal at the Eocene-Oligocene boundary, Clim. Past, 7, 235–247, https://doi.org/10.5194/cp-7-235-2011, 2011. a, b
Timmermann, A., Okumura, Y., An, S.-I., Clement, A., Dong, B., Guilyardi, E., Hu, A., Jungclaus, J., Renold, M., Stocker, T. F., Stouffer, R., Sutton, R., Xie, S.-P., and Yin, J.: The influence of a weakening of the Atlantic meridional overturning circulation on ENSO, J. Clim., 20, 4899–4919, 2007. a, b, c
Timmermann, A., An, S.-I., Kug, J.-S., Jin, F.-F., Cai, W., Capotondi, A., Cobb, K. M., Lengaigne, M., McPhaden, M. J., Stuecker, M. F., Stein, K., Wittenberg, A. T., Yun, K.-S., Bayr, T., Chen, H.-C., Chikamoto, Y., Dewitte, B., Dommenget, D., Grothe, P., Guilyardi, E., Ham, Y.-G., Hayashi, M., Ineson, S., Kang, D., Kim, S., Kim, W., Leet, J.-Y., Li, T., Lua, J.-J., McGregor, S., Planton, Y., Power, S., Rashid, H., Ren, H.-L., Santoso, A., Takahashi, K., Todd, A., Wang, G., Wang, G., Xie, R., Yang, W.-H., Yeh, S.-W., Yoon, J., Zeller, E., and Zhang, X.: El Niño–southern oscillation complexity, Nature, 559, 535–545, 2018. a
Toumoulin, A., Tardif, D., Donnadieu, Y., Licht, A., Ladant, J.-B., Kunzmann, L., and Dupont-Nivet, G.: Evolution of continental temperature seasonality from the Eocene greenhouse to the Oligocene icehouse –a model–data comparison, Clim. Past, 18, 341–362, https://doi.org/10.5194/cp-18-341-2022, 2022. a
van Kampenhout, L., Rhoades, A. M., Herrington, A. R., Zarzycki, C. M., Lenaerts, J. T. M., Sacks, W. J., and van den Broeke, M. R.: Regional grid refinement in an Earth system model: impacts on the simulated Greenland surface mass balance, The Cryosphere, 13, 1547–1564, https://doi.org/10.5194/tc-13-1547-2019, 2019. a
Van Nes, E. H., Scheffer, M., Brovkin, V., Lenton, T. M., Ye, H., Deyle, E., and Sugihara, G.: Causal feedbacks in climate change, Nat. Clim. Change, 5, 445–448, 2015. a
Vannitsem, S., Dalaiden, Q., and Goosse, H.: Testing for dynamical dependence: Application to the surface mass balance over Antarctica, Geophys. Res. Lett., 46, 12125–12135, 2019. a
Veron, J. E., Hoegh-Guldberg, O., Lenton, T. M., Lough, J. M., Obura, D. O., Pearce-Kelly, P., Sheppard, C. R., Spalding, M., Stafford-Smith, M. G., and Rogers, A. D.: The coral reef crisis: The critical importance of <350 ppm CO2, Mar. Pollut. Bull., 58, 1428–1436, 2009. a, b
Vettoretti, G. and Peltier, W. R.: Thermohaline instability and the formation of glacial North Atlantic super polynyas at the onset of Dansgaard-Oeschger warming events, Geophys. Res. Lett., 43, 5336–5344, 2016. a, b
Vettoretti, G., Ditlevsen, P., Jochum, M., and Rasmussen, S. O.: Atmospheric CO2 control of spontaneous millennial-scale ice age climate oscillations, Nat. Geosci., 15, 300–306, 2022. a
Via, R. K. and Thomas, D. J.: Evolution of Atlantic thermohaline circulation: Early Oligocene onset of deep-water production in the North Atlantic, Geology, 34, 441–444, 2006. a
Voigt, A. and Marotzke, J.: The transition from the present-day climate to a modern Snowball Earth, Clim. Dynam., 35, 887–905, 2010. a
Wang, G., Cai, W., Gan, B., Wu, L., Santoso, A., Lin, X., Chen, Z., and McPhaden, M. J.: Continued increase of extreme El Niño frequency long after 1.5 ∘ warming stabilization, Nat. Clim. Change, 7, 568–572, 2017. a
Wang, S., Foster, A., Lenz, E. A., Kessler, J. D., Stroeve, J. C., Anderson, L. O., Turetsky, M., Betts, R., Zou, S., Liu, W., Boos, W. R., and Hausfather, Z.: Mechanisms and impacts of Earth system tipping elements, Rev. Geophys., 61, e2021RG000757, https://doi.org/10.1029/2021RG000757, 2023. a, b, c
Wang, X., Auler, A. S., Edwards, R. L., Cheng, H., Cristalli, P. S., Smart, P. L., Richards, D. A., and Shen, C.-C.: Wet periods in northeastern Brazil over the past 210 kyr linked to distant climate anomalies, Nature, 432, 740–743, 2004. a
Wassenburg, J. A., Vonhof, H. B., Cheng, H., Martínez-García, A., Ebner, P.-R., Li, X., Zhang, H., Sha, L., Tian, Y., Edwards, R. L., Fiebig, J., and Haug, G. H.: Penultimate deglaciation Asian monsoon response to North Atlantic circulation collapse, Nat. Geosci., 14, 937–941, 2021. a, b
Weaver, A. J., Saenko, O. A., Clark, P. U., and Mitrovica, J. X.: Meltwater pulse 1A from Antarctica as a trigger of the Bølling-Allerød warm interval, Science, 299, 1709–1713, 2003. a
Weertman, J.: Stability of the junction of an ice sheet and an ice shelf, J. Glaciol., 13, 3–11, 1974. a
Weijer, W., Cheng, W., Drijfhout, S. S., Fedorov, A. V., Hu, A., Jackson, L. C., Liu, W., McDonagh, E., Mecking, J., and Zhang, J.: Stability of the Atlantic Meridional Overturning Circulation: A review and synthesis, J. Geophys. Res.-Ocean., 124, 5336–5375, 2019. a, b, c
Wengel, C., Lee, S.-S., Stuecker, M. F., Timmermann, A., Chu, J.-E., and Schloesser, F.: Future high-resolution El Niño/Southern Oscillation dynamics, Nat. Clim. Change, 11, 758–765, 2021. a, b, c
Westerhold, T., Marwan, N., Drury, A. J., Liebrand, D., Agnini, C., Anagnostou, E., Barnet, J. S. K., Bohaty, S. M., Vleeschouwer, D. D., Florindo, F., Frederichs, T., Hodell, D. A., Holbourn, A. E., Kroon, D., Lauretano, V., Littler, K., Lourens, L. J., Lyle, M., Pälike, H., Röhl, U., Tian, J., Wilkens, R. H., Wilson, P. A., and Zachos, J. C.: An astronomically dated record of Earth’s climate and its predictability over the last 66 million years, Science, 369, 1383–1387, 2020. a
Willeit, M., Ganopolski, A., Robinson, A., and Edwards, N. R.: The Earth system model CLIMBER-X v1.0 – Part 1: Climate model description and validation, Geosci. Model Dev., 15, 5905–5948, https://doi.org/10.5194/gmd-15-5905-2022, 2022. a, b
Williamson, M. S., Collins, M., Drijfhout, S. S., Kahana, R., Mecking, J. V., and Lenton, T. M.: Effect of AMOC collapse on ENSO in a high resolution general circulation model, Clim. Dynam., 50, 2537–2552, 2018. a
Winkelmann, R., Donges, J. F., Smith, E. K., Milkoreit, M., Eder, C., Heitzig, J., Katsanidou, A., Wiedermann, M., Wunderling, N., and Lenton, T. M.: Social tipping processes towards climate action: a conceptual framework, Ecol. Econ., 192, 107242, https://doi.org/10.1016/j.ecolecon.2021.107242, 2022. a
Wolff, E. W., Chappellaz, J., Blunier, T., Rasmussen, S. O., and Svensson, A.: Millennial-scale variability during the last glacial: The ice core record, Quaternary Sci. Rev., 29, 2828–2838, 2010. a
Wood, R. A., Rodríguez, J. M., Smith, R. S., Jackson, L. C., and Hawkins, E.: Observable, low-order dynamical controls on thresholds of the Atlantic Meridional Overturning Circulation, Clim. Dynam., 53, 6815–6834, 2019. a
Wunderling, N., Willeit, M., Donges, J. F., and Winkelmann, R.: Global warming due to loss of large ice masses and Arctic summer sea ice, Nat. Commun., 11, 1–8, 2020. a, b, c, d
Wunderling, N., Donges, J. F., Kurths, J., and Winkelmann, R.: Interacting tipping elements increase risk of climate domino effects under global warming, Earth Syst. Dynam., 12, 601–619, https://doi.org/10.5194/esd-12-601-2021, 2021. a, b, c
Wunderling, N., Krönke, J., Wohlfarth, V., Kohler, J., Heitzig, J., Staal, A., Willner, S., Winkelmann, R., and Donges, J. F.: Modelling nonlinear dynamics of interacting tipping elements on complex networks: the PyCascades package, Eur. Phys. J.-Spec. Top., 230, 3163–3176, 2021b. a
Wunderling, N., Staal, A., Sakschewski, B., Hirota, M., Tuinenburg, O. A., Donges, J. F., Barbosa, H. M., and Winkelmann, R.: Recurrent droughts increase risk of cascading tipping events by outpacing adaptive capacities in the Amazon rainforest, P. Natl. Acad. Sci. USA, 119, e2120777119, https://doi.org/10.1073/pnas.2120777119, 2022. a
Wunderling, N., Winkelmann, R., Rockström, J., Loriani, S., Armstrong McKay, D. I., Ritchie, P. D., Sakschewski, B., and Donges, J. F.: Global warming overshoots increase risks of climate tipping cascades in a network model, Nat. Clim. Change, 13, 75–82, 2023. a, b, c, d
Zebiak, S. E. and Cane, M. A.: A model el niñ–southern oscillation, Mon. Weather Rev., 115, 2262–2278, 1987. a
Zemp, D. C., Schleussner, C.-F., Barbosa, H. M., Hirota, M., Montade, V., Sampaio, G., Staal, A., Wang-Erlandsson, L., and Rammig, A.: Self-amplified Amazon forest loss due to vegetation-atmosphere feedbacks, Nat. Commun., 8, 1–10, 2017. a
Zhang, N., Feng, M., Hendon, H. H., Hobday, A. J., and Zinke, J.: Opposite polarities of ENSO drive distinct patterns of coral bleaching potentials in the southeast Indian Ocean, Sci. Rep., 7, 2443, https://doi.org/10.1038/s41598-017-02688-y, 2017. a
Zhang, X., He, J., Zhang, J., Polyakov, I., Gerdes, R., Inoue, J., and Wu, P.: Enhanced poleward moisture transport and amplified northern high-latitude wetting trend, Nat. Clim. Change, 3, 47–51, 2013. a
Zhang, X., Lohmann, G., Knorr, G., and Purcell, C.: Abrupt glacial climate shifts controlled by ice sheet changes, Nature, 512, 290–294, 2014. a, b
Ziemen, F. A., Kapsch, M.-L., Klockmann, M., and Mikolajewicz, U.: Heinrich events show two-stage climate response in transient glacial simulations, Clim. Past, 15, 153–168, https://doi.org/10.5194/cp-15-153-2019, 2019. a
Zular, A., Sawakuchi, A. O., Chiessi, C. M., d’Horta, F. M., Cruz, F. W., Demattê, J. A. M., Ribas, C. C., Hartmann, G. A., Giannini, P. C. F., and Soares, E. A. A.: The role of abrupt climate change in the formation of an open vegetation enclave in northern Amazonia during the late Quaternary, Glob. Planet. Change, 172, 140–149, 2019. a
See https://tipmip.pik-potsdam.de/, last access: 14 January 2024.
- Abstract
- Introduction
- Interactions between climate tipping elements and nonlinear climate components
- Possible examples of interactions between tipping elements from a paleoclimatic perspective
- Modeling tipping element interactions and cascading transitions
- Discussion and conclusion
- Data availability
- Author contributions
- Competing interests
- Disclaimer
- Special issue statement
- Acknowledgements
- Financial support
- Review statement
- References
- Supplement
- Article
(3453 KB) - Full-text XML
- Abstract
- Introduction
- Interactions between climate tipping elements and nonlinear climate components
- Possible examples of interactions between tipping elements from a paleoclimatic perspective
- Modeling tipping element interactions and cascading transitions
- Discussion and conclusion
- Data availability
- Author contributions
- Competing interests
- Disclaimer
- Special issue statement
- Acknowledgements
- Financial support
- Review statement
- References
- Supplement