the Creative Commons Attribution 4.0 License.
the Creative Commons Attribution 4.0 License.
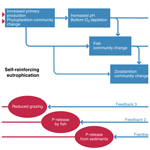
Lake ecosystem tipping points and climate feedbacks
Tom Andersen
David Armstrong McKay
Sarian Kosten
Mariana Meerhoff
Amy Pickard
Bryan M. Spears
Lakes and ponds experience anthropogenically forced changes that may be non-linear and sometimes initiate ecosystem feedbacks leading to tipping points beyond which impacts become hard to reverse. In many cases climate change is a key driver, sometimes in concert with other stressors. Lakes are also important players in the global climate by ventilating a large share of terrestrial carbon (C) back to the atmosphere as greenhouse gases and will likely provide substantial feedbacks to climate change. In this paper we address various major changes in lake ecosystems and discuss if tipping points can be identified, predicted, or prevented, as well as the drivers and feedbacks associated with climate change. We focus on potential large-scale effects with regional or widespread impacts, such as eutrophication-driven anoxia and internal phosphorus (P) loading, increased loading of organic matter from terrestrial to lake ecosystems (lake “browning”), lake formation or disappearance in response to cryosphere shifts or changes in precipitation to evaporation ratios, switching from nitrogen to phosphorus limitation, salinization, and the spread of invasive species where threshold-type shifts occur. We identify systems and drivers that could lead to self-sustaining feedbacks, abrupt changes, and some degree of resilience, as opposed to binary states not subject to self-propelling changes or resilience. Changes driven by warming, browning, and eutrophication can cause increased lake stratification, heterotrophy (browning), and phytoplankton or macrophyte mass (eutrophication), which separately or collectively drive benthic oxygen depletion and internal phosphorus loading and in turn increase greenhouse gas (GHG) emissions. Several of these processes can feature potential tipping point thresholds, which further warming will likely make easier to surpass. We argue that the full importance of the vulnerability of lakes to climate and other anthropogenic impacts, as well as their feedback to climate, is not yet fully acknowledged, so there is a need both for science and communication in this regard.
- Article
(1425 KB) - Full-text XML
- BibTeX
- EndNote
In natural sciences, the hysteretic behaviour of lakes (Scheffer and van Nes, 2007) has informed the concept of tipping points at the ecosystem level, following the development of the alternative stable state theory in shallow lakes (Scheffer et al., 1993). Given the global vulnerability of freshwaters and the pervasive nature of major pressures acting upon them (e.g. nutrient pollution, over-extraction, and climate change), tipping points in these systems could have significant societal impacts, including on human and environmental health, clean water and food production, and climate regulation. The capacity to detect discontinuous ecosystem responses to pressure changes in natural systems has been challenged (e.g. Hillebrand et al., 2020; Davidson et al., 2023). Nevertheless, there are several studies that have reported the occurrence of tipping points even if they are difficult to detect (Lade et al, 2021; Seekell et al., 2022), such as shifts from one alternative state to another in small shallow lakes, the most common lake type globally (Messager et al., 2016).
Some types of changes can be classified as binary, i.e. either-or situations at the system level. Increased temperature and/or reduced precipitation may induce negative water balance and shrinking of water volumes to the level where lakes or ponds simply disappear. Many lakes worldwide are facing reduced water volumes, but perhaps most striking is the widespread loss of high-latitude waterbodies, from Arctic or sub-Arctic ponds to wetlands or bogs. Such phenomena may qualify as one type of tipping point and are not self-propelled by internal feedbacks per se but rather by higher evaporation-to-precipitation ratios (Smol and Douglas, 2007) or permafrost thaw (Smith et al., 2005; Webb et al., 2022; Smol, 2023). While most tundra ponds and other small waterbodies hardly qualify as lakes (Richardson et al., 2022), we mostly use the word lake through the text for simplicity, yet it will be evident from the context of wording where we specifically refer to ponds.
The question of what constitutes a “sudden” system shift, alternative stable states, and hysteresis also depends on what is regarded as a relevant time span: days, years, decades, or centuries. Also, systems may have alternative states that are not necessarily fixed over long time spans; hence the phrase “stable” should be used with caution, and the term “hysteresis” should infer little from the strength of the regulating processes. Uncertainty also remains on the geographical extent of tipping points in lakes and the wider relevance for the Earth's climate system. Single lakes or local areas may experience non-linear or abrupt changes caused by local drivers, but we focus here on potential tipping points of global or regional relevance and with relevance to the climate system.
Empirical analyses, process modelling, and experimental studies are advanced for shallow lakes, providing a good understanding of lake ecosystem behaviour around tipping points. There are related concepts in the literature (regime shifts, catastrophic shifts, forward switches, etc.), and there are clearly many aspects of abrupt changes in nature and society that could be labelled tipping points (Carrier-Belleau et al., 2022), but here we adopt the definition of a tipping point occurring when self-sustaining change in a system is triggered beyond a forcing threshold, typically starting with positive feedback loops, then entering a runaway phase before the tipping point finally brings the system into a different alternative state (van Nes et al., 2016; Lenton et al., 2023). For example, the well-documented increase in phosphorus (P) loading across European lakes in the last century (e.g. from agricultural and wastewater pollution) has uncovered critical loading thresholds beyond which lakes can shift rapidly from a clear-water, submerged-macrophyte-rich state to a turbid, phytoplankton-dominated state (Scheffer et al., 2001; Tátrai et al., 2008) and vice versa when nutrient loading decreases. One of the theoretical implications is that to induce a switch back to the initial state the nutrient loading should be reduced to a lower threshold before the shift might be possible (hysteresis). Adding to such well-described and mechanistically well-understood changes, there is a range of phenomena that may be perceived as tipping points. Hence, to provide structure to this complexity, a range of tipping point candidates should be scrutinized against a common assessment approach. To qualify as tipping points, phenomena should not just be isolated phenomena in single lakes but be more general and hold for specific (and widespread) types of lakes or waterbodies. Such phenomena may thus in the future occur across geographically distinct lake populations experiencing similar environmental change. In this way, the potential for identifying regional- or global-scale changes can be framed (Fig. 1).
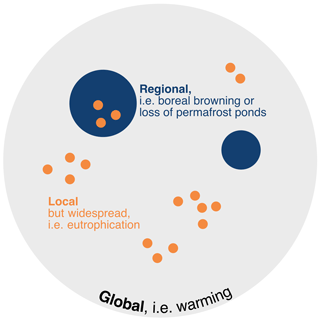
Figure 1Impacts at levels that may qualify for tipping points at relevant spatial scales. Regional or biome-wise effects could be loss of ponds and lakes due to permafrost thaw and/or increased loadings of DOM in the boreal biome or salinization. Also, local but widespread changes such as anthropogenic eutrophication of lakes in populated or intense agricultural areas would have large-scale impacts. Lakes worldwide show a warming trend, hence a global impact.
It is well-established that lakes are sensitive to the effects of climate change, including warming and changes in precipitation and storminess (e.g. Adrian et al., 2009; Meerhoff et al., 2022). Emerging evidence suggests that lakes and ponds may also play an important role in climate regulation, through both the emission of greenhouse gases (i.e. CO2 and CH4, predominantly CH4; Downing et al., 2021) and carbon (C) burial (Anderson et al., 2020). Lakes and rivers are impacted by climate change and other anthropogenic pressures globally, but they also provide strong feedbacks to the global climate systems and carbon cycle (Cole et al., 2007; Tranvik et al., 2009), despite comprising a small part of the global water extent. While global estimates of net greenhouse gas (GHG) emissions from lakes remain poorly constrained, there is general consensus that a significant fraction of terrestrially fixed C is degassed to the atmosphere via surface waters. Cole et al. (2007) conservatively estimated that inland waters annually receive some 1.9 Pg C yr−1 from the terrestrial landscape, of which at least 0.8 Pg C yr−1 is returned to the atmosphere through water-to-atmosphere GHG exchange. Later estimates revised this global GHG exchange term, to include evasion rates, to 2.1 Pg C yr−1 from lakes, rivers, and reservoirs (Raymond et al., 2013). Notably, boreal lakes are important conduits of CO2 release to the atmosphere, estimated to be equivalent to the annual CO2 release from forest fires globally (Hastie et al., 2017). Under a high-CO2-emission scenario and as a result of increased terrestrial net primary production (NPP), CO2 emissions from boreal lakes are projected to increase by 107 %, showing the coupling between the terrestrial and aquatic C cycle (Hastie et al., 2017).
This significant role of surface waters for GHG emissions is also highly relevant, but poorly constrained, in both national and global C budgets (Lindroth and Tranvik, 2021). The relationship between inputs of organic C and nutrients is a key determinant of the balance between heterotrophic and autotrophic processes, determining the biodiversity, community composition, and food web structure, as well as the productivity-to-respiration (P : R) ratio. And so, it is relevant to consider the extent to which potential tipping points may drive, or be driven by, climate change, leading to higher-level feedbacks to the Earth's climate system.
Here, we discuss candidate tipping points in freshwaters reported in the literature (based on literature searches including the term “tipping point” and either “lake” or “pond”) and the experience and insights of the author team. The discussion on each is constrained to waterbody categories with the potential for global or at least regional or biome-scale relevance. In this context we also constrain the discussion to potential tipping points that are more generic, at least carrying regional or biome-wide impact, and that could have feedbacks to the climate while not necessarily being driven or triggered by climate change per se.
We identify six candidate categories for tipping points at a relevant scale in this context (regional to global impact), and for each of the categories we discuss whether observed changes can be categorized as tipping points according to the definition above. We also address climatic and other drivers and consequences, including potential feedbacks to the climate system, and wider societal implications, with emphasis on the most relevant and influential categories.
In principle, any abrupt or sudden stress imposed on a waterbody could result in specific impacts, i.e. toxic waste or toxic treatments (e.g. rotenone to kill off undesired species and runoff of herbicides inadvertently killing aquatic plants), hydrological alterations by impoundment or canals, and stocking or immigration of new (often exotic) species. In some cases, when stressors are removed the system will return abruptly to its original state. Here, for a phenomenon to qualify as a tipping point, we require that system response should be self-sustaining and involve positive feedbacks, in line with the criteria set out in van Nes et al. (2016) and Lenton et al. (2023). To be relevant in a wider context, the tipping point should be more generic to certain types of impact and certain types of waterbodies and potentially also have feedbacks to the climate in terms of GHG emissions. We have identified six stressors that may trigger a freshwater ecosystem to cross a tipping point (Table 1) and scrutinize them in turn below.
Table 1Candidate events from the literature with potential to occur at local to regional scales, their association with climate change, and whether tipping points and hystereses have been associated with them. Parentheses indicate higher uncertainty.
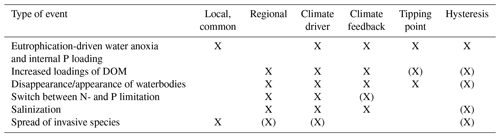
2.1 Eutrophication, anoxia, and internal P loading
Eutrophication is one of the most pervasive stressors on freshwater and coastal systems. Although it may naturally occur due to inputs from the watershed or from biota translocating nutrients from connecting ecosystems, eutrophication is a largely human-induced phenomenon. The main causes of cultural eutrophication have varied across time and regions. However, it is widely accepted that the current main cause of eutrophication is the change in land use in watersheds, particularly agricultural activities driving diffuse nutrient pollution (and other agrochemicals) (Moss, 2008; Schulte-Uebbing et al., 2022). Agriculture, with myriad impacts on freshwaters that go well beyond nutrient pollution (Moss, 2008), has been identified as a major driver of ecosystem shifts and tipping points (Gordon et al., 2008).
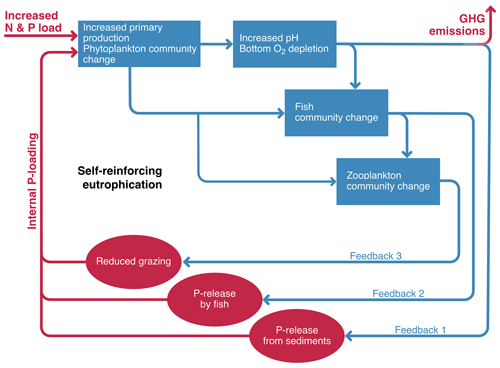
Figure 2Feedback loop diagram for eutrophication, demonstrating key feedbacks that can amplify P loading and beyond a tipping point cause self-sustaining change. Eutrophication and internal mobilization of P cause high algal biomass, decreased benthic oxygen or anoxia, and consequently also increased greenhouse gas emissions. Blue rectangles denote primary responses, and red circles denote the secondary feedback responses and the key driver and consequence (nutrient loading and GHG release).
The mobilization of P from lake bed sediments, a process known as internal loading (Sondergaard et al., 2001), plays a key role in hysteresis in lakes following the reduction in P loading from the catchment (Boström et al., 1982; Jeppesen et al., 1991; Spears and Steinman, 2020). In this context, hysteresis can be strengthened by eutrophication-driven biological changes in fish composition and size structure that have cascading effects on zooplankton and phytoplankton and strong impacts on fish-mediated nutrient cycling (Brabrand et al., 1990). This in turn will maintain a system with deep-water anoxia and high nutrient load, supporting the release of GHGs (Fig. 2).
Feedbacks and tipping points
The phenomenon of eutrophication is local, but widespread, and likely to worsen in its manifestations as a result of climate change (Moss et al., 2011; Meerhoff et al., 2022). In particular, the process of internal loading may be enhanced by lake warming (Jeppesen et al., 2009) due to the increased metabolism of bacteria and an acceleration of biochemical reactions. Warming also increases stratification and the duration and strength of stratification, also promoting anoxia (Maberly et al., 2020; Woolway et al., 2020, 2022). As a case example, this phenomenon is well-documented by the recent study of the shallow and highly eutrophic Danish lake, Ormstrup (Davidson et al., 2024). Increases in precipitation and high-intensity rainfall events are also expected to significantly increase runoff of P from agricultural catchments to surface freshwaters (Ockenden et al., 2017), further promoting eutrophication and its manifestations.
The different states of shallow lakes can feed back differently on climate by either reducing or increasing GHG emissions (Hilt et al., 2017). Clear and turbid lakes differ in their CO2 emissions due to the magnitude of CO2 uptake by primary-producer photosynthesis. Efflux of CO2 appears to decrease when submerged macrophytes establish after the reduction in nutrient loading (Jeppesen et al., 2016). Shallow, submerged-macrophyte-dominated lakes tend to emit lower CH4 by ebullition and diffusion than turbid, phytoplankton-dominated lakes (Colina et al., 2022; Davidson et al., 2018). The turbid state in particular feeds back on climate since warming and eutrophication-induced water anoxia could offset increased CO2 fixation by blooms or by macrophytes as lower oxygen levels stimulate methane (CH4) emissions, with CH4 emissions from eutrophic systems expected to increase by 6 %–20 % with each degree of warming (Aben et al., 2017).
The eutrophication- and warming-associated shift from submerged macrophyte dominance to phytoplankton or floating plant dominance may also strongly increase greenhouse gas emissions, particularly CH4 (Aben et al., 2022). Cyanobacterial blooms, a typical manifestation of eutrophication and high internal P loading, can both promote CO2 sequestration and produce CH4. CH4 can be produced even under oxic conditions as a by-product of photosynthesis (Bižić et al., 2020). Blooms often create anoxic layers in surface sediments or through the water column after their collapse, favouring the production and transport of CH4 via methanogenesis (Li et al., 2021; Yan et al., 2017). Cyanobacterial blooms are thus considered a key mechanism by which eutrophication has a positive feedback on climate change (Bižić, 2021; Yan et al., 2017). Although increased inputs on nitrogen (N) from atmospheric deposition or catchment runoff are the main causes of elevated N2O release from lakes (Yang et al., 2015), warming also impacts aquatic N2O emissions. N2O emissions are estimated to increase by 8 %–14 % for each degree of warming (Velthuis and Veraart 2022), highlighting another strong climate feedback.
Despite the fact that nutrient loading is still the major driver of eutrophication (including algal blooms; e.g. Bonilla et al., 2023), climate change is also expected to promote eutrophication (Moss et al., 2011; Meerhoff et al., 2022). Indeed, interaction between temperature and trophy has been observed to produce synergistic emission responses in experimental lakes (Davidson et al., 2018), and warming alters resident microbial communities to favour methanogenesis over methanotrophy (Zhu et al., 2020). It is thus likely that warming decreases the nutrient thresholds for a tipping point leading to a shift to an alternative state in shallow lakes and ponds.
The predominantly amplifying influence of climate change on eutrophication-driven tipping points in lakes provides a mechanism for coherent threshold exceedance beyond the local scale, with more widespread eutrophication-induced tipping points expected with further warming. However, despite the dearth of studies that generate bi-directional carbon flux data to assess the balance between emission and burial in lakes, it remains unknown whether the climate feedback can be buffered by projected eutrophication-driven increases in lake carbon burial (Anderson et al., 2020). Moreover, robust projections are lacking for climate impacts on eutrophication, with no emergent regional-to-global warming threshold identifiable beyond which a non-linear increase in these localized tipping points occurs (Grasset et al., 2020). In general, tipping points become harder to predict in a warmer climate (Kosten et al., 2009).
2.2 Increased loadings of DOM in the boreal biome
Over thousands to millions of years, the feedback between terrestrial vegetation and aquatic productivity has been essential for the evolution of the atmosphere and the global climate (Beerling, 2008). Vegetation serves not only as a major C pool and eventually a source of total organic carbon (TOC) in boreal areas, but it also promotes root exudates of CO2 and organic C. This enhances weathering rates, thereby increasing the flux of nutrients (P, N, Si, Fe, Ca, and carbonate (CO3)) (Humborg et al., 2004; Hessen et al., 2009) to surface waters. The availability of nutrients subsequently enhances aquatic productivity and thereby C sequestration. In addition, the carbonate species are important for buffering capacity towards acidification in freshwater and marine systems. On different timescales there is thus a range of feedback mechanisms between terrestrial and aquatic ecosystems that demand a better understanding. Tracking past-history (Holocene) tree lines, forest cover, and lake sediments revealed a strong and consistent link between climate, forest cover, and lake TOC (Rühland et al., 2003; Rosén, 2005). Thus, at least on the centennial scale, there is a strong temporal TOC link between terrestrial and aquatic systems. Allochthonous C derived either directly as leachate from litterfall and roots or indirectly via partial decomposition of organic matter in the soils constitutes (by far) the dominant pool of dissolved organic matter (DOM) in boreal freshwaters; hence, forest cover and the fraction of bogs and wetland areas in the catchment are key determinants for the concentration and colour of this terrestrially derived chromophoric DOM (Dillon and Molot, 2005; Larsen et al., 2011a).
Since terrestrially derived C is a main determinant of freshwater C, any changes in terrestrial primary production and export of organic C will invariably also increase aquatic outputs of CO2. Increased terrestrial productivity has been linked to “CO2 fertilization” (Huang et al., 2007), yet these CO2 effects will be constrained by N availability. Elevated N deposition due to human emissions has driven an increase in the forest C sink in tandem with the CO2 fertilization effect while also increasing the deficiency in P (and other key elements allocated to tree biomass) (De Vries et al., 2006).
Increased export of terrestrially derived DOM to lakes and rivers in boreal regions (“browning”) is a widespread phenomenon partly linked to reduced acidification but also driven by land-use changes (notably afforestation) and climate change (CO2 fertilization of forests, warming, and hydrology) (de Wit et al., 2016; Creed et al., 2018; Monteith et al., 2023). An empirically based space-for-time model of changes in the Normalized Difference Vegetation Index (NDVI) under a 2 °C climate scenario predicts continued browning of boreal lakes (Larsen et al., 2011b). Forest dynamics are slow, however; hence, space-for-time scenarios projecting increased flux of TOC from catchments owing to increased forest cover could require centuries to play out. Thus, catchment properties governing production of TOC such as forest size and the fraction of bog and wetland areas could very well be temporally decoupled from the export, especially considering the large stock of organic matter typically present in boreal catchments.
Time series analysis (30 years) of data from 70 Norwegian catchments and lakes provided evidence for a tight temporal coupling between the decadal increase in land “greening” (with NDVI as a proxy) and lake browning (with TOC as a proxy) (Finstad et al., 2016), and the browning on northern lakes can to a large extent be attributed to recent afforestation (Kritzberg, 2017; Skerlep et al., 2020). The prominent “greening” by increased vegetation cover trend in many boreal and alpine regions (Guay et al., 2014) and increase in forest volume (Opdahl et al., 2023) will thus have bearings on lakes and rivers in these regions. There are a number of confounding explanatory drivers for this greening: warming, elevated CO2, accumulated nitrogen deposition, and changes in grazing activities, as well as forestry practices, are all implicated. An extended growing season has also been recorded (Barichivicz et al., 2017), and elevated levels of CO2 per se may contribute to this (Piao et al., 2006). Collectively, these changes in environmental drivers and pressures yield an increase in terrestrial net primary production. Since a significant fraction of the terrestrial NPP will be exported to surface waters as DOM, it means that terrestrial greening could lead to freshwater browning.
The role of forest cover is further accentuated by a need for a carbon-negative future (i.e. net drawdown of CO2 from the atmosphere) where widespread afforestation is the only currently feasible means of reducing atmospheric concentrations of CO2 beyond the continued action of natural carbon sinks (MacDougall et al., 2020). However, such afforestation also comes with climate costs, both in terms of decreased albedo (Betts and Ball, 1997; Bathiany et al., 2010; Lawrence et al., 2022) and, as argued above, the potential for increased production and degassing of GHGs from surface waters. Enhanced primary production in forested catchments stimulated by reactive N deposition has, by increasing the pool of C available for fluvial export, been linked to increased C burial in northern lakes over the past 2 centuries (Heathcote et al., 2015). Again, this highlights the need for improved understanding of the balance between C emissions and burial in lakes in response to browning (Williamson et al., 2015) and other identified stressors in order to better constrain climate feedbacks. Browning will also promote darkening of coastal waters with as yet unknown climate feedbacks (Opdal et al., 2023). The question remains whether these terrestrial and aquatic responses are directly coupled in time or if there is a delayed aquatic response in the order of decades or even millennia. Another question is how the CO2 in itself could boost these processes and how this skewed C supply to autotrophs could affect land-aquatic interactions.
Wide-scale shifts in boreal lakes caused by increased loadings of DOM can promote a prolonged and more intensified stratification period (implications summarized above, described for DOM by Spears et al., 2017), amplified by warming. Increased terrestrial DOM loadings intensify net heterotrophy in the systems (i.e. through increased light attenuation and increased access to organic C for heterotrophic bacteria) (Hessen et al., 1990; Karlsson et al., 2007; Thrane et al 2014; Horppila et al., 2023). A well-explored case study as an example of these impacts, which is also linked directly to the tipping point concept, is the boreal brown-water Swedish lake, Härsvatten, where a long-term study clearly links loadings of DOC to anoxia (Spears et al., 2017). While at present the thresholds around these effects have not been well-constrained, the impacts may be significant at the global scale for GHG emissions (Tranvik et al., 2009) and regionally for coastal primary producers (Opdal et al., 2023). Given the strong empirical links between drivers and consequences, impacts and feedbacks can be predicted qualitatively, while not yet quantitatively.
The temporal aspect also deserves further attention. If the main source of browning is afforestation, responses will proceed slowly compared with cases where reduced acid deposition is the main driver, yet both drivers operate on decadal timescales. In the latter case, the browning could represent a re-browning (Meyer-Jacob et al., 2020).
Feedbacks and tipping points
The links and feedbacks between climate to land to lakes and back to climate in terms of increased GHG emissions is conceptually well-understood, and the main drivers for the specific GHGs (CO2, CH4 and N2O) in boreal areas are also understood (Yang et al., 2015; Wik et al., 2016; Valiente et al., 2022). However, the question of whether these feedbacks can result in tipping points by becoming self-sustaining beyond a threshold is not yet settled. Most boreal lakes are net heterotrophic and thus conduits of CO2, often also CH4, due to high concentrations of DOM and common deep-water anoxia. A shift from net autotrophy to net heterotrophy would classify as a binary shift, yet with a strong positive climate feedback. If it eventually led to oxygen depletion and cascading feedbacks, then it would qualify as a tipping point. However, there would be a time delay between the two events, with the latter being the critical tipping event. There is also a commonly reported unimodal response in lakes to increased loadings of DOM, typically around 5 mg DOC L−1 (Karlsson et al., 2007; Thrane et al., 2014), where increases in DOM below the threshold may promote NPP and thus CO2 drawdown due to N and P associated with DOM, while reduced NPP and increased degassing of CO2 (and CH4) will take place above. We thus propose two types of large-scale potential tipping points, one related to anoxia and the other to DOM-concentrations, yet both are related to increasing load of terrestrially derived DOM across the boreal region.
2.3 Disappearance/appearance of waterbodies
A global reduction in lake-water storage (Pekel et al., 2016; Yao et al., 2023) and the climate-driven creation or disappearance of waterbodies are crucial issues. Loss of waterbodies due to overuse, warming, or drought pose a major threat to vulnerable freshwater resources, also by deteriorating water quality or salinization (cf. below). The most dramatic warming has already taken place in the high Arctic with temperature increases up to 3 °C over the past few decades (Wang et al., 2022), further promoting the onset of permafrost thaw (Langer et al., 2016). Current and future permafrost thaw and glacier melting can both create new waterbodies and drain old ones, providing a strong link to the fate of the cryosphere (Smith et al., 2005; Olefeldt et al., 2021). Such small, but numerous, waterbodies residing on permafrost over large geographical scales in Eurasia and North America are currently among the most vulnerable waterbodies globally (Smol and Douglas, 2007). They host species-poor but specific communities of invertebrates (Walseng et al., 2018) of vital importance for bird life and other biota. Warming may also affect these waterbodies indirectly via glacier melt, increased inputs of organic C, fertilization by increasing populations of geese (caused by climate change), and consequently changes in microbial communities and increased GHG emissions (Wei et al., 2023). Thus, by their sheer number, these systems may also serve as increasingly important conduits of GHGs and historical soil carbon stocks to the atmosphere (Negandhi et al., 2016) and play an important role in mediating nutrient delivery to the polar oceans (Emmerton et al., 2008), potentially affecting global NPP (Terhaar et al., 2021). While the main problem is the loss of waterbodies affected by warming-induced increased evapotranspiration rates (Smol and Douglas, 2007) and permafrost thaw (Smith et al., 2005), there are also cases where collapsing palsas and thermokarst areas create new waterbodies, and these waterbodies may themselves represent a positive feedback by accelerating the thaw (Langer et al., 2016; Turetsky et al., 2020).
Since most of these potentially lost waterbodies are small and nameless ponds, it is hard to point to specific cases, but the works cited above provide a number of telling examples. While the focus in this context is negative water balance or the loss of high-latitude waterbodies, this is actually a widespread problem causing the shrinking of many lakes. In Arctic areas, responses to warming may differ substantially between perennial lakes and ephemeral wetlands, related to ambient temperature and permafrost depth. Although the loss or gain of waterbodies is not classified as a tipping event in a very strict sense, i.e. there are not obvious strong, self-reinforcing factors involved, it is still a climate-driven change with potentially large, widespread, and irreversible consequences and with repercussions on climate in terms of changes in GHG emissions from vast areas.
Feedbacks and tipping points
Some essential feedbacks to climate change are involved in the change in Arctic waterbodies; e.g. reduced ice and snow cover in the Arctic will promote further permafrost thaw. Certain Arctic areas have experienced a major increase in breeding birds, notably geese, that promote increased loadings of organic C and nutrients (Hessen et al., 2017). More organic carbon entering waterbodies from their terrestrial surroundings, combined with warming and eventually bird-induced eutrophication, promotes GHG emissions (Wei et al., 2023). It is important to make clear that some of the impacts are contrasting; i.e. the loss of waterbodies may at first increase GHG emissions (Keller et al., 2020; Paranaiba et al., 2021) but will eventually reduce GHG emissions. Permafrost thaw and drainage of water-logged areas will increase CO2 emissions but could reduce CH4 emissions. The sudden release of methane hydrates upon permafrost thaw is a possibility, yet it is hard to predict and quantify and is not specifically linked to aquatic habitats.
Few changes are as irreversible as complete habitat loss, and the climate-driven loss of numerous waterbodies residing on permafrost over large geographical scales in Eurasia and North America (due to permafrost thaw) with climate feedbacks in terms of changed GHG emissions is possible. In fact, as argued by Smol and Douglas (2007), “the final ecological threshold for these aquatic ecosystems has now been crossed: complete desiccation”. If strictly adhering to the tipping point criteria as an event occurring when self-sustaining change in a system is triggered beyond a forcing threshold, typically starting with positive feedback loops and a runaway phase before the tipping point finally brings the system into a different alternative state, the loss of waterbodies is not strictly a tipping point but a binary shift. Abrupt permafrost thaw, which can drive abrupt self-sustained formation or draining of thermokarst lakes, is categorized as a “regional impact” climate-tipping element by Armstrong McKay et al. (2022). We extend this categorization to include the lakes associated with these abrupt thaw processes, seeing them as coupled permafrost–lake systems with tipping dynamics involving both components (Turetsky et al., 2020). Despite the scale considered here, the extent of open water globally is relatively easy to quantify using remote sensing, and it is possible to make predictions based on time series and empirical relationships between temperature increase, permafrost thaw, and the loss of waterbodies. Quantifying potential climate feedbacks related to the processing of organic C to CO2 and CH4 should be possible to predict within orders of magnitude, with initial analysis suggesting that abrupt thaw involving thermokarst lake formation and draining could double the warming impact of gradual permafrost thaw (Turetsky et al., 2020).
2.4 Switch from N- to P limitation
Imbalance in biogeochemical cycles has become a major concern both on the local and global scale. Anthropogenic emissions of CO2 now appear as the major environmental challenge for ecosystems and human wellbeing in the foreseeable future. In relative terms, however, the anthropogenic effects on the global N cycle are even more pronounced. Transformation of atmospheric N2 to more reactive reduced or oxidized forms of inorganic N by the fertilizer industry and combustion processes has dramatically changed. Recent analyses of the global N cycle (Bodirsky et al., 2014; Zhang et al., 2020) suggest that various human activities currently convert similar N2 to total natural ecosystem fixation and that the use of both N and P are far beyond “safe boundaries” (Rockström et al., 2023).
Increased N deposition may affect surface waters in fundamentally different ways. It will increase the emissions of N2O (Yang et al., 2015), and the increased deposition of inorganic N promotes soil and water acidification through increased NO3 in surface waters. It will, however, also affect elemental ratios in lakes and rivers (Hessen et al., 2009). The relative proportions of these elements will determine the nature of elemental limitation for both autotrophs and a range of heterotrophs and could thus profoundly affect community composition and ecosystem processes. One effect of such skewed inputs of N over P would be an intensified P limitation in surface waters or even large-scale shifts from N- to P limitation (Elser et al., 2009).
Rather than pinpointing specific lakes as examples, contrasting areas with high versus low N deposition, Elser et al. (2009) provide good regional examples, where Colorado offers examples of regions with either high or low N deposition, while southern regions of Norway and Sweden experience up to 10-fold elevated levels of N deposition compared with central regions.
Conversely, increased N loss by denitrification, eventually associated with increased internal P loading, may shift systems from P- to N limitation (Weyhenmeyer et al., 2007). Societal implications include an increased prevalence in toxin-producing cyanobacteria, purported to be promoted to an extent by warming (Paerl et al., 2008) and favouring non-N-fixing toxin-producing species where reduced N concentrations are high relative to oxidized N (Hoffman et al., 2022). Additionally, a threshold on toxic effects on sensitive freshwater species has been proposed (i.e. 2 mg L−1; Camargo et al., 2006; Moss et al., 2013), above which a marked decline in biodiversity is expected.
Feedbacks and tipping points
Changes in N- versus P limitation of NPP are associated with changes in community structure, both for the phytoplankton and macrophyte communities. While the shift from one limiting nutrient to another represents a binary shift and abrupt transition, it is not driven by self-propelling events or positive or negative feedbacks, since a shift from N- to P limitation is typically caused by N deposition or agricultural use of fertilizers. While increased N loading per se could promote climate feedbacks in terms of N2O, the switch from N- to P limitation or vice versa is neither driven by climate nor exhibits strong feedbacks on climate. There is also no inherent hysteresis, and, when drivers change, the system may immediately return to the other limiting nutrient. For these reasons we do not classify this category as a tipping point according to the definition above.
2.5 Salinization
Salinization is a prevalent threat to freshwater rivers, lakes, and wetlands worldwide, particularly in arid and semi-arid regions and coastal areas. It is caused by a range of anthropogenic actions including water extraction, pollution, and climate change (Herbert et al., 2015). The causes of salinization have historically been classified as being primary or secondary. Primary salinization refers to natural causes including wet and dry deposition of marine salts, weathering of rocks, and surface or groundwater flows transporting salts from geological salt deposits. Secondary salinization refers to salinization caused by human activities such as irrigation with water rich in salts, rising of brackish and saline groundwater due to increased groundwater extraction, and increased seawater intrusion as a result of sea level rise. The distinction between natural and anthropogenic causes underlying salinization is becoming less clear-cut due to climate change as anthropogenically caused changes in temperature, precipitation patterns, and wind will affect the primary salinization processes (Oppenheimer et al., 2019). Salinization has severe consequences for aquatic communities (Jeppesen et al., 2015; Cunillera-Montcusí et al., 2022). Salinization has a strong ecological impact often associated with osmotic stress and changes in biogeochemical cycles which often entails an increase in concentration of toxic sulfides (Herbert et al., 2015). In addition, studies focussing on the application of road salts indicate that salinization may disrupt lake-water mixing and the release of metals (Szklarek et al., 2022 and references therein). Negative effects of increased salinity have been described for trophic levels ranging from microorganisms to fish and birds (reviewed by Cunillera-Montcusí et al., 2022). In addition, salinization also has a high societal impact particularly related to domestic and agricultural water supply in arid and semi-arid regions (Williams et al., 1999).
A strong example of climate-driven salinization and its impact on biota is the long-term study (1938–2004) in two Canadian lakes (Sereda et al., 2011). Concomitant with periodic declines in precipitation, lake elevation declined and salinity increased in the Jackfish and Murray lakes from 1938 to 2004. The increase in salinity caused an estimated 30 % loss in diversity of macrobenthos. If salinity exceeds thresholds where key species or functional groups are wiped out, it will no doubt represent an abrupt ecosystem transition, yet still not a tipping point in the sense that it is self-propelled.
Feedbacks and tipping points
Regime shifts from clear to turbid may occur at 6–8 ‰ salinity in systems with intermediate to high nutrient loadings and have been associated with a change in zooplankton community composition from cladocerans to more salinity-tolerant cyclopoid copepods (Jeppesen et al., 2007). Salinity-induced regime shifts may also lead to dominance by microbial mats at the expense of submerged macrophytes (Davis et al., 2003; Sim et al., 2006). While there are species-specific tolerance thresholds to salinity, and these effects are expected to interact with other stressors – including eutrophication (Jeppesen et al., 2007; Kaijser et al., 2019), colour, and turbidity (Davis et al., 2003) – the process is not driven by feedbacks of increased salinization but by external factors like warming, water (over)use, and road salting. Hysteresis after refreshing of salinized systems has been little studied but is likely strongly biogeochemical in nature as evidenced by previously brackish waters that have been flushed with freshwater for over 90 years and still contain high levels of chloride, sodium, and sulfate (van Dijk et al., 2019).
A weakened top-down control by zooplankton on phytoplankton occurring at moderately high salinities would be an indirect consequence of salinization, leading to a worsening of eutrophication symptoms (Gutierrez et al., 2018) and thus promoting indirect climate effects.
Salinization, however, tends to decrease CH4 emissions (Herbert et al., 2015; Chamberlain et al., 2020; Gremmen et al., 2022). The decrease in CH4 emissions can either be caused by a decrease in CH4 production – e.g. because methanogens are outcompeted by sulfate reducers or are negatively impacted by sulfide toxicity – or because of an increase in methane oxidation (reviewed by Herbert et al., 2015). The salinity-induced decrease in aquatic CH4 emissions may imply a negative feedback with climate change but only when this is not offset by a decrease in carbon burial. Insight into this balance is currently limited (Chamberlain et al., 2020), and, while there is no doubt salinization is widespread on regional scales and may reach threshold values for species and processes, we do not categorize it as a tipping point under the cited criteria.
2.6 Spread of invasive species
Freshwaters are especially vulnerable to species loss and population declines, as well as species invasions, due to their constrained spatial extent. Substantial ecosystem changes by reinforcing interactions between invasive species and alternative states (i.e. macrophyte versus phytoplankton dominance, as described above) may occur (Reynolds and Aldridge, 2021). The spread of several invasive species can change community composition and ecological functions in dramatic ways and can be regarded as sudden transitions with major site-specific or regional impacts. Moreover, species invasions can be facilitated by climate change (Rahel and Olden, 2008), and, notably, flooding and other hydrological events can facilitate species invasion with potentially far-reaching ecological consequences (Anufriieva and Shadrin, 2018).
There are numerous examples of ecological consequences in lakes following species invasions, and the major impacts of invasions by zebra mussel and the predatory cladoceran Bythotrephes in the Great Lakes serve as striking examples of major impacts at the regional scale even in very large lakes (Ricciardi and MacIsaac, 2000). While species invasions are of major ecological and societal concern and can induce ecological tipping points in certain lakes, they are generally not self-perpetuating involving internal feedbacks. There is no doubt it may be appropriate to say that the invasion of a system may cause irreversible changes or hysteresis in specific lakes or lakes within regions.
Feedbacks and tipping points
Climate, both in the context of warming that opens the latitudinal and altitudinal spread of species (Hessen et al., 2006) and of hydrological events that likewise may promote invasions (Anufriieva and Shadrin, 2018), may pose drastic changes in community composition and ecosystem functions to an extent that qualifies as abrupt shifts. Species invasions may also interact with other drivers, lowering the potential thresholds (of nutrients, temperature, browning, etc.) for a shift to occur, and vice versa, by impacting on previously occurring stabilizing mechanisms (Willcock et al., 2023). Likewise, species shifts may have repercussions on GHG emissions. We do not pursue the discussion feedbacks and potential tipping points further for this candidate category, however, since we have constrained our definition of tipping points to situations with internal feedback and regional occurrence. Given the widespread anthropogenic changes promoting invasive species in aquatic communities worldwide, the often abrupt and unpredictable shifts that may follow from this deserve further attention.
Freshwaters are one of the most vulnerable ecosystems and resources globally and will increasingly be so in the warming world. They also link catchment properties and terrestrial changes to marine systems, and, notably, lakes serve as good sentinels of global change (Adrian et al., 2009). Population declines and species loss in freshwaters are happening at an alarming pace, underpinning the urgency for evidence of ecological tipping points in response to environmental change. Drinkable freshwater is a scarce resource both in terms of water quality and availability (Yao et al., 2023). Predicting (and preventing) sudden shifts in water quality and quantity is therefore also a high priority from an anthropocentric perspective, and insights into feedbacks, thresholds, and tipping points are highly relevant to lakes. Lakes are also major players in the global climate, and, besides being highly vulnerable to climate change, they can provide strong feedback to the climate by ventilating a substantial share of terrestrially fixed C back to the atmosphere as CO2 and CH4 (Cole et al., 2007; Tranvik et al., 2009; Raymond et al., 2013). Lakes are also subject to changes, sometimes sudden, due to climate change and other natural or anthropogenic drivers. In fact, some of the first and most striking examples on tipping points and regime shift come from lake studies (Scheffer et al., 1993).
We argue that there are two key drivers that may shift lakes towards major ecological changes and increased climate feedback by GHG emissions, namely eutrophication and browning. Both these drivers are promoted by warming, which may be seen as a separate driver. Both processes are also characterized to some degree by self-sustaining feedback loops, feed back to climate in terms of GHG emissions, and are also strongly integrated with land surface impacts in the catchment (Fig. 3). Warming, browning, and eutrophication lead to increases in stratification, heterotrophy, and phytoplankton or macrophyte mass, which collectively drive benthic oxygen depletion and, in turn, increased GHG emissions (helping to drive further warming and DOM loading from land) and internal P loading (driving further eutrophication) (Meerhoff et al., 2022). Several of these processes can feature tipping points (eutrophication and potentially DOM loading), which warming will likely make easier to reach.
Few processes have been more thoroughly described in terms of drivers, impacts, and remedies than freshwater eutrophication. The drivers are well-known (nutrient loadings from agricultural activities and locally also from sewage discharges), despite long-term controversies regarding the relative importance of N or P in promoting eutrophication (e.g. Smith and Schindler, 2009; Paerl et al., 2016). There are also long traditions for predictive hydraulic models that link the load of P to algal blooms and benthic O2 depletion (e.g. Vollenweider-type models; Imboden, 1974). Moreover, given the scarcity, increasing demands and increasing prices of P as a commodity worldwide, there are indeed strong arguments to close the loop for P and reduce losses to the environment (Brownlie et al., 2022). Due to the strong impact of O2 depletion on sediment release of P and thus internal fertilization (Soendergaard et al., 2001), which will play in concert with food-web-driven feedbacks (cf. Fig. 3), tipping points in this context can be identified, while the climate component is difficult to separate.
Browning shares many of these attributes in terms of increased net heterotrophy. The shift from net autotrophy with a net uptake of CO2 to net heterotrophy with a net release of CO2 (plus CH4) also represents a binary situation. However, since most boreal lakes are already net heterotrophic owing to microbial conversion of organic C (Hessen et al., 1990; Cole et al., 1994; Larsen et al., 2011c), most boreal lakes simply become more heterotrophic; hence there is no tipping point in this context. However, an increased degree of heterotrophy, combined with increased thermal stability, will promote deep-water anoxia and thereby internal P cycling and GHG release. Since the key driver here is the external load of terrestrial DOM, the feedback component driving P release is weaker than in the case of eutrophication. Nevertheless, an increased release of GHGs no doubt poses a feedback to the climate and hence to the terrestrial systems that may promote further browning. These processes are amplified by climate change and have global consequences in terms of GHG emissions. Given the high confidence in this case, we recommend it as a priority for the parametrization of models to underpin future predictions of the impacts of global tipping points in lakes on GHG emissions.
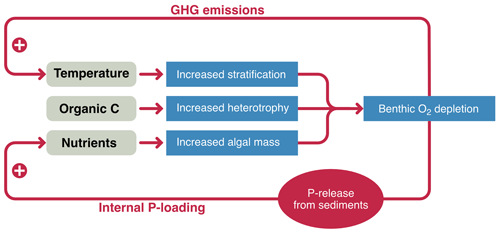
Figure 3The interactive role of eutrophication, DOM export (browning), and warming on lakes. Separately or combined, they promote benthic O2 depletions which cause an internal feedback by P loading from sediments and a climate feedback via the release of greenhouse gases. The potential shift between states (blue rectangles to red circle) is indicated.
As a separate type of binary tipping point which is likely to be widespread and related to GHG release, we propose the loss of waterbodies, notably Arctic ponds. This is driven by permafrost thaw in the case of thermokarst-linked lake formation or disappearance (categorized as a regional tipping element in previous assessments (Armstrong McKay et al., 2022). Together, the coupled permafrost–lake system can act as a localized tipping system with the lake providing key feedbacks to help drive self-sustaining thaw. This makes the tipping points easy to monitor (by remote sensing) and predictable in the sense that they will be closely linked to permafrost thaw. There are, however, feedbacks to the climate, with potentially high emissions during the drying process (Marcé et al., 2019; Turetsky et al., 2020). A different situation would be the less-widespread case of new waterbodies formed by collapsing palsas in cases of retreating glaciers. Given the potential scale of occurrence, the net effect of permafrost thaw and increased release of CO2 balanced with the effect of disappearing waterbodies and potential changes in net GHG emissions requires further attention as a matter of high priority.
One could argue that what matters is whether a change or process is linear (and thus more predictable) or non-linear (and less predictable) and that the rest is semantics. This is not truly the case, since there are substantial differences in what is regarded as a “tipping point”, not least in terms of whether impacts are easily reversible or are effectively “locked in” (e.g. hysteresis). Still, from an ecosystem perspective, abrupt shifts, even if they do not qualify as tipping points, may have devastating effects that should urge us to invest more in preventing deterioration as we do not know where/if a sudden shift may occur. As argued by Moss et al. (2008), “the sort of precision demanded by legislators and lobbies will never be attainable, and this has been a major weapon used to delay regulation of agricultural activities”.
Shifts between ecological states do not necessarily involve alternative stable states with hysteresis. In fact, the concepts of both abruptness and irreversibility depend on one's perspective of time. Over a lake's lifetime, shifts back and forth between states occurring over years or even decades are “sudden” relative to the human lifespan. For example, Rühland et al. (2008) report apparent coherence in diatom community shifts post-1850 on hemispheric scales over 100 years or so. Similarly, a coherent global increase in hypoxia in lakes has been reported over a 100-year period (from about 1850) by Jenny et al. (2016). Likewise, regional patterns of species turnover (β diversity) over 200 years demonstrated regional differences in species turnover but also recent changes attributed to warming (Kahlert et al., 2020). As warming progresses, such studies form good baselines for future changes. If the observational time step is increased to centuries, then it is likely that more large-scale examples will come through in palaeo-studies. In fact, there are several examples of coherence in lake responses to climate variability or climate change, some of which take place over short time spans (Stone et al., 2016; Isles et al., 2023). Finally, multiple drivers may jointly push lakes towards shifts or tipping points, as shown in Huang et al. (2022) and Willcock et al. (2023).
Taken together, there are at least two major reasons why an improved understanding of sudden changes in lake ecosystems is imperative: they are highly vulnerable to climate change and other anthropogenic stressors globally, and they serve as major feedbacks to the climate system through GHG emissions. Being well-mixed and semi-closed entities that reflect changes in catchment properties, they also serve as sentinels of global change (Adrian et al., 2019). For freshwaters in general, lakes are crucial in the hydrological cycling, and they link the terrestrial and marine ecosystems. The major tipping point dynamics converge in oxygen depletion, primarily in deeper strata and the sediment surface, which promotes feedbacks and hysteresis in terms of internal P release, as well as increased GHG emissions. High nutrient load, increased inputs of dissolved organic C, and warming all drive oxygen depletion, and, while many problems related to global warming boil down to the obvious recommendation of reduced use of fossil fuel and other GHG-emitting activities, reducing nutrient use and losses to within the carrying capacity of the system (i.e. from ecosystem- to global scales) is comparatively simpler for both N and P when compared to C (Rockström et al., 2009, 2023). The incentives should be even greater for closing the P loop, given the potential for scarcity of this non-substitutable element and its role in lake eutrophication (Brownlie et al., 2022).
Regime shifts and tipping points are concepts closely linked to resilience (Andersen et al., 2008; Spears et al., 2017). Lakes represent excellent model case studies in this respect and have been widely used to demonstrate theories of ecological stability and resilience that are needed to underpin preventative management approaches and to guide science-based environmental policy. The full importance of the vulnerability of lakes to climate and other anthropogenic impacts, as well as their feedback to climate, is not yet fully acknowledged, so there is a need for both science and communication in this regard. However, we argue that the search for empirical evidence to underpin theory should not prevent societies and managers taking more action to protect freshwaters in the meantime.
Anthropogenic forcing may induce non-linear, abrupt changes in freshwater ecosystems, and awareness of such potential threshold effects is important. Here, we focus on lake and pond systems that may be subject to tipping points, where self-reinforcing feedback and some type of post-change hysteresis can be identified. To qualify in this context, these changes must also be relevant on larger scales (i.e. regions or biomes) or in a large number of systems. Two types of potential tipping points were identified based on these criteria: eutrophication and browning. The first is an example of a widespread phenomenon, with the second occurring in lakes in the boreal biome. In both cases, climate is involved as a driver, and the changes in terms of deep-water anoxia and internal P cycling may boost the emission of GHGs from such systems. While not tipping points according to the criteria applied here, two types of binary shifts are also discussed: loss of waterbodies, notably in the Arctic areas, caused by permafrost thaw or negative water balance, and shifts between N- and P limitation caused by N deposition. Notably, the first is driven by climate change and will also have repercussions on climate by changes in GHG emissions. Finally, salinization and species invasions are also changes that may occur on large scales with potentially abrupt ecosystem changes, with changes in temperature and precipitation as important drivers.
No data sets were used in this article.
DOH conceived the idea and wrote the first draft. All authors contributed to the writing and approved the final paper. TA, DAM, SK, MM, AM, and BMS all provided inputs on various parts of the text, and all authors also provided suggestions to the selection and ranking of tipping elements, suggested examples and references and read, commented and approved the final version of the paper.
The contact author has declared that none of the authors has any competing interests.
Publisher’s note: Copernicus Publications remains neutral with regard to jurisdictional claims made in the text, published maps, institutional affiliations, or any other geographical representation in this paper. While Copernicus Publications makes every effort to include appropriate place names, the final responsibility lies with the authors.
This article is part of the special issue “Tipping points in the Anthropocene”. It is a result of the “Tipping Points: From Climate Crisis to Positive Transformation” international conference hosted by the Global Systems Institute (GSI) and University of Exeter (12–14 September 2022), as well as the associated creation of a Tipping Points Research Alliance by GSI and the Potsdam Institute for Climate Research, Exeter, Great Britain, 12–14 September 2022.
This work has benefitted from discussions within the group behind the Tipping Point report presented at COP28 meeting in Dubai, and the paper has benefitted substantially from inputs and suggestions by John Smol and an anonymous reviewer on the first draft.
This paper was edited by Jonathan Donges and reviewed by John Smol and one anonymous referee.
Aben, R. C. H., Barros, N., van Donk, E., Frenken, T., Hilt, S., Kazanjian, G., Lamers, L. P. M., Peeters, E. T. H. M., Roelofs, J. G. M., de Senerpont Domis, L. N., Stephan, S., Velthuis, M., Van de Waal, D. B., Wik, M., Thornton, B. F., Wilkinson, J., DelSontro, T., and Kosten, S.: Cross continental increase in methane ebullition under climate change, Nat. Comm., 8, 1682, https://doi.org/10.1038/s41467-017-01535-y, 2017.
Aben, R. C. H., Velthuis, M., Kazanjian, G., Frenken, T., Peeters, E. T. H. M., Van de Waal, D. B., Hilt, S., de Senerpont Domis, L. N., Lamers, L. P. M., and Kosten, S.: Temperature response of aquatic greenhouse gas emissions differs between dominant plant types, Water Res., 226, 119251, https://doi.org/10.1016/j.watres.2022.119251, 2022.
Adrian, R., O'Reilly, C. M., Zagarese, H., Baines, S. B., Hessen, D. O., Keller, W., Livingstone, D. M., Sommaruga, R., Straile, D., Van Donk, E., and Weyhenmeyer, G. A.: Lakes as sentinels of climate change, Limnol. Oceanogr., 54, 2283–2297, https://doi.org/10.4319/lo.2009.54.6_part_2.2283, 2009.
Andersen, T., Carstensen, J., Hernández-Garcia, E., and Duarte, C. M.: Ecological thresholds and regime shifts: approaches to identification, Trends Ecol. Evol., 24, 49–52, https://doi.org/10.1016/j.tree.2008.07.014, 2008.
Anderson, N. J., Heathcote, A. J., Engstrom, D. R., et al.: Anthropogenic alteration of nutrient supply increases the global freshwater carbon sink, Sci. Adv., 6, 2145, https://doi.org/10.1126/sciadv.aaw2145, 2020.
Anufriieva, E. V. and Shadrin, N. V.: Extreme hydrological events destabilize aquatic ecosystems and open doors for alien species, Quaternary Int., 475, 11–15, https://doi.org/10.1016/j.quaint.2017.12.006, 2018.
Armstrong McKay, D. I., Staal, A., Abrams, J. F., Winkelmann, R., Sakschewski, B., Loriani, S., Fetzer, I., Cornell, S. E., Rockström, J., and Lenton, T. M.: Exceeding 1.5 °C global warming could trigger multiple climate tipping points, Science, 377, eabn7950, https://doi.org/10.1126/science.abn7950, 2022.
Barichivicz, J., Briffa, K. R., Mynemi, R. B., et al.: Large-scale variations in the vegetation growing season and annual cycle of atmospheric CO2 at high northern latitudes from 1950 to 2011, Glob. Change Biol., 19, 3167–3183, 2017.
Bathiany, S., Claussen, M., Brovkin, V., Raddatz, T., and Gayler, V.: Combined biogeophysical and biogeochemical effects of large-scale forest cover changes in the MPI earth system model, Biogeosciences, 7, 1383–1399, https://doi.org/10.5194/bg-7-1383-2010, 2010.
Beerling, D.: The Emerald Planet, Oxford Press, 2008.
Bižić, M.: Phytoplankton photosynthesis: an unexplored source of biogenic methane emission from oxic environments, J. Plank. Res., 43, 822–830, https://doi.org/10.1093/plankt/fbab069, 2021.
Betts, A. K. and Ball, J. H.: Albedo over the boreal forest, J. Geophys. Res., 102, 28901–28909, https://doi.org/10.1029/96JD03876, 1997.
Bižić, M., Klintzsch, T., Ionescu, D., Hindiyeh, M.Y., Günthel, M., Muro-Pastor, A. M., Eckert, W., Urich, T., Keppler, F., and Grossart, H. P.: Aquatic and terrestrial cyanobacteria produce methane, Sci. Adv., 6, eaax5343, https://doi.org/10.1126/sciadv.aax5343, 2020.
Bodirsky, B., Popp, A., Lotze-Campen, H., et al.: Reactive nitrogen requirements to feed the world in 2050 and potential to mitigate nitrogen pollution, Nat. Commun., 5, 3858, https://doi.org/10.1038/ncomms4858, 2014.
Bonilla, S., Aguilera, A., Aubriot, L., Huszar, V., Almanza, V., Haakonsson, S., Izaguirre, I., O'Farrell, I., Salazar, A., Becker, V., and Cremella, B.: Nutrients and not temperature are the key drivers for cyanobacterial biomass in the Americas, Harmful Algae, 121, 102367, https://doi.org/10.1016/j.hal.2022.102367, 2023.
Boström, B., Jansson, M., and Forsberg, C. Phosphorus release from lake sediments, Arch. Hydrobiol. Beih. Ergebn. Limnol., 18, 5–59, 1982.
Brabrand, Å., Faafeng, B. A., and Nilssen J. P. M.: Relative Importance of Phosphorus Supply to Phytoplankton Production: Fish Excretion versus External Loading, Can. J. Fish. Aquat. Sci., 47, 364–372, https://doi.org/10.1139/f90-038, 1990.
Brownlie, W. J., Sutton, M. A., Heal, K. V., Reay, D. S., and Spears, B. M. (Eds.): Our Phosphorus Future, UK Centre for Ecology and Hydrology (UKCEH), Edinburgh, https://doi.org/10.13140/RG.2.2.17834.08645, 2022.
Camargo, J. A., Alonso, A., and Salamanca, A.: Nitrate toxicity to aquatic animals: a review with new data for freshwater invertebrates, Chemosphere, 58, 1255–1267, https://doi.org/10.1016/j.chemosphere.2004.10.044, 2005.
Carrier-Belleau, C., Pascal, L., Nozais, C., and Archambault, P.: Tipping points and multiple drivers in changing aquatic ecosystems: A review of experimental studies, Limnol. Oceanogr., 67, S312–S330, https://doi.org/10.1002/lno.11978, 2022.
Chamberlain, S. D., Hemes, K. S., Eichelmann, E., Szutu, D. J., Verfaillie, J. G., and Baldocchi, D. D.: Effect of Drought-Induced Salinization on Wetland Methane Emissions, Gross Ecosystem Productivity, and Their Interactions, Ecosystems, 23, 675–688, https://doi.org/10.1007/s10021-019-00430-5, 2020.
Cole, J. J., Caraco, N., Kling, G., and Kratz, T. K.: Carbon Dioxide Supersaturation in the Surface Waters of Lakes, Science, 265, 1568–1570, https://doi.org/10.1126/science.265.5178.1568, 1997.
Colina, M., Kosten, S., Silvera, N., Clemente, J. M., and Meerhoff, M.: Carbon fluxes in subtropical shallow lakes: contrasting regimes differ in CH4 emissions, Hydrobiologia, 849, 3813–3830, https://doi.org/10.1007/s10750-021-04752-1, 2021.
Creed, I., Bergström, A. K., Trick, C. B., et al.: Global change-driven effects on dissolved organic matter composition: Implications for food webs of northern lakes, Glob. Change Biol., 24, 3692–3714, https://doi.org/10.1111/gcb.14129, 2018.
Cunillera-Montcusí, D., Beklioğlu, M., Cañedo-Argüelles, M., et al.: Freshwater salinisation: a research agenda for a saltier world, Trend. Ecol. Evol., 37, 440–453, 2022.
Davis, J. A., McGuire, M., Halse, S. A., Hamilton, D., Horwitz, P., McComb, A. J., Froend, R. H., Lyons, M., and Sim, L.: What happens when you add salt: predicting impacts of secondary salinisation on shallow aquatic ecosystems by using an alternative-states model, Austr. J. Bot., 51, 715–724, 2003.
Davidson, T. A., Audet, J., Jeppesen, E., Landkildehus, F., Lauridsen, T. L., Søndergaard, M., and Syväranta, J.: Synergy between nutrients and warming enhances methane ebullition from experimental lakes, Nat. Clim. Change, 8, 156–160, https://doi.org/10.1038/s41558-017-0063-z, 2018.
Davidson, T. A., Sayer, C. D., Jeppesen, E., et al.: Bimodality and alternative equilibria do not help explain long-term patterns in shallow lake chlorophyll-a, Nat. Comm., 14, 398, https://doi.org/10.1038/s41467-023-36043-9, 2023.
Davidson, T. A., Søndergaard, M., Audet, J., Levi, E., Espositio, C., and Nielsen, A.: Temporary stratification promotes large greenhouse gas emissions in a shallow eutrophic lake, Biogeosciences, 21, 93–107, https://doi.org/10.5194/bg-21-93-2024, 2024.
De Vries, W., Reinds, G.J., Gundersen, P., and Sterba, H.: The impact of nitrogen deposition on carbon sequestration in European forests and forest soils, Glob. Change Biol., 12, 1151–1173, https://doi.org/10.1111/j.1365-2486.2006.01151.x, 2006.
de Wit, H., Valinia, S., Weyhenmeyer, G., et al.: Current browning of surface waters will be further promoted by wetter climate, Environ. Sci. Technol. Lett., 12, 430–435, https://doi.org/10.1021/acs.estlett.6b00396, 2016.
Dillon, P. J. and Molot, L. A.: Long-term trends in catchment export and lake retention of dissolved organic carbon, dissolved organic nitrogen, total iron and total phosphorus: The Dorset, Ontario, study, 1978–1998, J. Geophys. Res.-Biogeo., 110, G01002, https://doi.org/10.1029/2004JG000003, 2005.
Downing, J. A., Polasky, S., Olmstead, S. M., and Newbold, S. C.: Protecting local water quality has global benefits, Nat. Comm., 12, 2709, https://doi.org/10.1038/s41467-021-22836-3, 2021.
Elser, J. J., Andersen, T., Baron, J., et al.: Shifts in Lake N : P Stoichiometry and Nutrient Limitation Driven by Atmospheric Nitrogen Deposition, Science, 326, 835–837, https://doi.org/10.1126/science.1176199, 2009.
Emmerton, C. A., Lesack, L. F. W., and Vincent, W. F.: Mackenzie River nutrient delivery to the Arctic Ocean and effects of the Mackenzie Delta during open water conditions, Global Biogeochem. Cy., 22, GB1024, https://doi.org/10.1029/2006GB002856, 2008.
Finstad, A., Andersen, T., Larsen, S., et al.: From greening to browning: Catchment vegetation development and reduced S-deposition promote organic carbon load on decadal time scales in Nordic lakes, Sci. Rep., 6, 31944, https://doi.org/10.1038/srep31944, 2016.
Gordon, L. J., Peterson, G. D., and Bennett, E. M.: Agricultural modifications of hydrological flows create ecological surprises, Trends Ecol. Evol., 23, 211–219, https://doi.org/10.1016/j.tree.2007.11.011, 2008.
Grasset, C., Sobek, S., Scharnweber, K., et al.: The CO2-equivalent balance of freshwater ecosystems is non-linearly related to productivity, Glob. Change Biol., 26, 5705–5715, https://doi.org/10.1111/gcb.15284, 2020.
Gremmen, T., van Dijk, G., Postma, J., Colina, M., de Senerpont Domis, L. N., Velthuis, M., van de Haterd, R., Kuipers, F., van Rossum, H., Smolders, A. J., and Kosten, S.: Factors influencing submerged macrophyte presence in fresh and brackish eutrophic waters and their impact on carbon emissions, Aquat. Bot., 187, 103645, https://doi.org/10.1016/j.aquabot.2023.103645, 2023.
Guay, K. C., Beck, P. S. A., Berner, L. T., Goetz, S. J., Baccini, A., and Buermann, W.: Vegetation productivity patterns at high northern latitudes: a multi-sensor satellite data assessment, Glob. Change Biol., 20, 3147–3158, https://doi.org/10.1111/gcb.12647, 2014.
Gutierrez, M. F., Tavşanoğlu, Ü.N., Vidal, N., Yu, J., Teixeira-de Mello, F., Çakiroglu, A. I., He, H., Liu, Z., and Jeppesen, E.: Salinity shapes zooplankton communities and functional diversity and has complex effects on size structure in lakes, Hydrobiologia, 813, 237–255, https://doi.org/10.1007/s10750-018-3529-8, 2018.
Hastie, A., Lauerwald, R., Weyhenmeyer, G., Sobek, S., Verpoorter, C., and Regnier, P.: CO2 evasion from boreal lakes: Revised estimate, drivers of spatial variability, and future projections, Glob. Change Biol., 24, 711–728, https://doi.org/10.1111/gcb.13902, 2017.
Heathcote, A., Anderson, N., Prairie, Y., Engstrom, D. R., and del Giorgio, P. A.: Large increases in carbon burial in northern lakes during the Anthropocene, Nat. Commun., 6, 10016, https://doi.org/10.1038/ncomms10016, 2015.
Herbert, E. R., Boon, P., Burgin, A. J., Neubauer, S. C., Franklin, R. B., Ardón, M., Hopfensperger, K. N., Lamers, L. P. M., and Gell, P.: A global perspective on wetland salinization: ecological consequences of a growing threat to freshwater wetlands, Ecosphere, 6, 1–43, https://doi.org/10.1890/ES14-00534.1, 2015.
Hessen, D. O. and Andersen, T.: Carbon metabolism in a humic lake: Pool sizes and cycling through zooplankton, Limnol. Oceanogr., 35, 84–99, https://doi.org/10.4319/lo.1990.35.1.0084, 1990.
Hessen, D. O., Andersen, T., Larsen, S. Skjelkvåle, B. L., and de Wit, H.: Nitrogen deposition, catchment productivity, and climate as determinants of lake stoichiometry, Limnol. Oceanogr., 54, 2520–2528, https://doi.org/10.4319/lo.2009.54.6_part_2.2520, 2009.
Hessen, D. O., Faafeng, B. A., Smith, V. H. Bakkestuen, V., and Walseng, B.: Extrinsic and intrinsic controls of zooplankton diversity in lakes, Ecology, 87, 433–443, https://doi.org/10.1890/05-0352, 2006.
Hessen, D. O., Tombre, I. M., van Geest, G., and Alfsnes, K.: Global change and ecosystem connectivity: How geese link fields of central Europe to eutrophication of Arctic freshwaters, Ambio, 46, 40–47, https://doi.org/10.1007/s13280-016-0802-9, 2017.
Hillebrand, H., Donohue, I., Harpole, W. S., et al.: Thresholds for ecological responses to global change do not emerge from empirical data, Nat. Ecol. Evol., 4, 1502–1509, https://doi.org/10.1038/s41559-020-1256-9, 2020.
Hilt, S., Brothers, S., Jeppesen, E., Veraart, A. J., and Kosten, S.: Translating regime shifts in shallow lakes into changes in ecosystem functions and services, BioScience, 67, 928–936, https://doi.org/10.1093/biosci/bix106, 2017.
Hoffman, D. K., McCarthy, M. J., Boedecker, A. R., Myers, J. A., and Newell, S. E.: The role of internal nitrogen loading in supporting non-N-fixing harmful cyanobacterial blooms in the water column of a large eutrophic lake, Limnol. Oceanogr., 67, 2028–2041, https://doi.org/10.1002/lno.12185, 2022.
Horppila, J., Keskinen, S., Nurmesniemi, M., Nurminen, L., Pippingsköld, E., Rajala, S., Sainio, K., and Estlander, S.: Factors behind the threshold-like changes in lake ecosystems along a water colour gradient: The effects of dissolved organic carbon and iron on euphotic depth, mixing depth and phytoplankton biomass, Freshw. Biol., 68, 1031–1040, https://doi.org/10.1111/fwb.14083, 2023.
Huang, J.-G., Bergeron, Y., Denneler, B., Berninger, F., and Tardif, J.: Response of Forest Trees to Increased Atmospheric CO2, Crit. Rev. Plant Sci., 26, 265–283, https://doi.org/10.1080/07352680701626978, 2007.
Huang, S., Zhang, K., Lin, Q., Liu, J., and Shen, J.: Abrupt ecological shifts of lakes during the Anthropocene, Earth-Sci. Rev., 227, 103981, https://doi.org/10.1016/j.earscirev.2022.103981, 2022.
Humborg, C., Smedberg, E., Blomqvist, S., et al.: Nutrient variations in subarctic Swedish rivers. Landscape control of land-sea fluxes, Limnol. Oceanogr., 49, 1871–1884, 2004.
Imboden, D. M.: Phosphorus model of lake eutrophication, Limnol. Oceanogr., 19, 297–304, https://doi.org/10.4319/lo.1974.19.2.0297, 1974.
Isles, P., Creed, I., Hessen, D. O., et al.: Widespread synchrony in phosphorus concentrations in northern lakes linked to winter temperature and summer precipitation, Limnol. Oceanogr. Lett., 8, 639–648, https://doi.org/10.1002/lol2.10318, 2023.
Jenny, J.-P., Francus, P., Normandeau, A., et al.: Global spread of hypoxia in freshwater ecosystems during the last three centuries is caused by rising local human pressure, Glob. Change Biol., 22, 1481–1489, https://doi.org/10.1111/gcb.13193, 2015.
Jeppesen, E., Kristensen, P., Jensen, J. P., Søndergaard, M., Mortensen, E., and Lauridsen, T.: Recovery resilience following a reduction in external phosphorus loading of shallow, eutrophic Danish lakes: duration, regulating factors and methods for overcoming resilience, Mem. Ist. Ital. Idrobiol., 48, 127–148, 1991.
Jeppesen, E., Søndergaard, M., Pedersen, A., Jürgens, K., Strzelczak, A., Lauridsen, T., and Johansson, L.: Salinity Induced Regime Shift in Shallow Brackish Lagoons, Ecosystems, 10, 48–58, https://doi.org/10.1007/s10021-006-9007-6 , 2007.
Jeppesen, E., Kronvang, B., Meerhoff, M., Søndergaard, M., Hansen, K. M., Andersen, H. E., Lauridsen, T. L., Beklioglu, M., Ozen, A., and Olesen, J. E.: Climate change effects on runoff, phosphorus loading and lake ecological state, and potential adaptations, J. Env. Qual., 38, 1930–1941, https://doi.org/10.2134/jeq2008.0113, 2009.
Jeppesen, E., Trolle, D., Davidson, T. A., Bjerring, R., Søndergaard, M., Johansson,L. S., Lauridsen, T. L., and Meerhoff, M.: Major changes in CO2 efflux when shallow lakes shift from a turbid to a clear water state, Hydrobiologia, 778, 33–44, https://doi.org/10.1007/s10750-015-2469-9, 2016.
Jeppesen, E., Brucet, S., Naselli-Flores, L., Papastergiadou, E., Stefanidis, K., Noges, T., Noges, P., Attayde, J. L., Zohary, T., and Coppens, J.: Ecological impacts of global warming and water abstraction on lakes and reservoirs due to changes in water level and related changes in salinity, Hydrobiologia, 750, 201–227, https://doi.org/10.1007/s10750-014-2169-x, 2015.
Kahlert, M., Rühland, K. M., Lavoie, I., et. al.: Biodiversity patterns of Arctic diatom assemblages in lakes and streams: Current reference conditions and historical context for biomonitoring, Freshw. Biol., 67, 116–140, https://doi.org/10.1111/fwb.13490, 2020.
Kaijser, W., Kosten, S., and Hering, D.: Salinity tolerance of aquatic plants indicated by monitoring data from the Netherlands, Aquat. Bot., 158, 103129, https://doi.org/10.1016/j.aquabot.2019.103129, 2019.
Keller, P. S., Catalán, N., von Schiller, D., et al.: Global CO2 emissions from dry inland waters share common drivers across ecosystems, Nat. Commun., 11, 2126, https://doi.org/10.1038/s41467-020-15929-y, 2020.
Kosten, S., Kamarainen, A., Jeppesen, E., van Nes, E. H., Peeters, E. T. H. M., Mazzeo, N., Sass, L., Hauxwell, J., Hansel-Welch, N., Lauridsen, T. L., Søndergaard, M., Bachmann, R. W., Lacerot, G., and Scheffer, M.: Climate-related differences in the dominance of submerged macrophytes in shallow lakes, Glob. Change Biol., 15, 2503–2517, https://doi.org/10.1111/j.1365-2486.2009.01969.x, 2009.
Kritzberg, E. S.: Centennial‐long trends of lake browning show major effect of afforestation, Limnol. Oceanogr. Lett., 2, 105–112, 2017.
Lade, S. J., Wang-Erlandsson, L., Staal, A., et al.: Empirical pressure-response relations can benefit assessment of safe operating spaces, Nat. Ecol. Evol., 5, 1078–1079, https://doi.org/10.1038/s41559-021-01481-5, 2021.
Langer, M., Westermann, S., Boike, J., Kirillin, G., Peng, S., and Krinner, G.: Rapid degradation of permafrost underneath waterbodies in tundra landscapes – Toward a representation of thermokarst in land surface models, JGR Earth Surf., 121, 2446–2470, https://doi.org/10.1002/2016JF003956, 2016.
Larsen, S., Andersen, T., and Hessen, D. O.: Predicting organic carbon in lakes from climate drivers and catchment properties, Global Biogeochem. Cy., 25, GB3007, https://doi.org/10.1029/2010GB003908, 2011a.
Larsen, S., Andersen, T., and Hessen, D. O.: Climate change predicted to cause severe increase of organic carbon in lakes, Glob. Change Biol., 17, 1186–1192, 2011b.
Larsen, S., Andersen, T., and Hessen, D. O.: The pCO2 in boreal lakes: Organic carbon as a universal predictor?, Global Biogeochem. Cy., 25, GB2012, https://doi.org/10.1029/2010GB003864, 2011c.
Lawrence, D., Coe, M., Walker, W., et al.: The Unseen Effects of Deforestation: Biophysical Effects on Climate, Front. Forest Glob. Change, 5, 756115, https://doi.org/10.3389/ffgc.2022.756115, 2022.
Lenton, T. M., Armstrong McKay, D. I., Loriani, S., Abrams, J. F., Lade, S. J., Donges, J. F., Milkoreit, M., Powell, T., Smith, S. R., Zimm, C., Buxton, J. E., Bailey, E., Laybourn, C., Ghadiali, A., and Dyke, J. G. (Eds.): 2023, The Global Tipping Points Report 2023, University of Exeter, Exeter, UK, https://global-tipping-points.org (last access: 17 April 2024), 2023.
Li, Y., Shang, J., Zhang, C., Zhang, W., Niu, L., Wang, L., and Zhang, H.: The role of freshwater eutrophication in greenhouse gas emissions: A review, Sci. Total Environ., 768, 144582, https://doi.org/10.1016/j.scitotenv.2020.144582, 2021.
Lindroth, A. and Tranvik, L.: Accounting for all territorial emissions and sinks is important for development of climate mitigation policies, Carbon Balance Mgm., 16, 10, https://doi.org/10.1186/s13021-021-00173-8, 2021.
Maberly, S. C., O'Donnell, R. A., Woolway, R. I., et al.: Global lake thermal regions shift under climate change, Nat. Commun., 11, 1232, https://doi.org/10.1038/s41467-020-15108-z, 2020.
MacDougall, A. H., Frölicher, T. L., Jones, C. D., Rogelj, J., Matthews, H. D., Zickfeld, K., Arora, V. K., Barrett, N. J., Brovkin, V., Burger, F. A., Eby, M., Eliseev, A. V., Hajima, T., Holden, P. B., Jeltsch-Thömmes, A., Koven, C., Mengis, N., Menviel, L., Michou, M., Mokhov, I. I., Oka, A., Schwinger, J., Séférian, R., Shaffer, G., Sokolov, A., Tachiiri, K., Tjiputra , J., Wiltshire, A., and Ziehn, T.: Is there warming in the pipeline? A multi-model analysis of the Zero Emissions Commitment from CO2, Biogeosciences, 17, 2987–3016, https://doi.org/10.5194/bg-17-2987-2020, 2020.
Marcé, R., Obrador, B., Gómez-Gener, L., Catalán, N., Koschorreck, M., Arce, M. I., Singer, G., and von Schiller, D.: Emissions from dry inland waters are a blind spot in the global carbon cycle, Earth-Sci. Rev., 188, 240–248, https://doi.org/10.1016/j.earscirev.2018.11.012, 2019.
Meerhoff, M., Audet, J., Davidson, T. A., De Meester, L., Hilt, S., Kosten, S., Liu, Z., Mazzeo, N., Paerl, H., Scheffer, M., and Jeppesen, E.: Feedback between climate change and eutrophication: revisiting the allied attack concept and how to strike back, Inland Waters, 12, 187–204, https://doi.org/10.1080/20442041.2022.2029317, 2022.
Messager, M., Lehner, B., Grill, G., et al.: Estimating the volume and age of water stored in global lakes using a geo-statistical approach, Nat. Commun., 7, 13603, https://doi.org/10.1038/ncomms13603, 2016.
Meyer-Jacob, C., Labaj, A. L., Paterson, A. M., Edwards, B. A., Keller, W., Cumming, B. F., and Smol, J. P.: Re-browning of Sudbury (Ontario, Canada) lakes now approaches pre-acidification lake-water dissolved organic carbon levels, Sci. Total Environ., 725, 138347, https://doi.org/10.1016/j.scitotenv.2020.138347, 2020.
Monteith., D. T., Henry, P. H. Hruska, J., et al.: Long-term rise in riverine dissolved organic carbon concentration is predicted by electrolyte solubility theory, Sci. Adv., 9, 3491, https://doi.org/10.1126/sciadv.ade3491, 2023.
Moss, B.: Water pollution by agriculture, Philos. T. R. Soc. B, 363, 659–666, https://doi.org/10.1098/rstb.2007.2176, 2008.
Moss B., Kosten, S., Meerhoff, M., Battarbee, R. W., Jeppesen, E., Mazzeo, N., Havens, K., Lacerot, G., Liu, Z., De Meester, L., Paerl, H., and Scheffer, M.: Allied attack: climate change and nutrient pollution, Inland Waters, 1, 101–105, https://doi.org/10.5268/IW-1.2.359, 2011.
Moss, B., Jeppesen, E., Søndergaard, M., et al.: Nitrogen, macrophytes, shallow lakes and nutrient limitation: resolution of a current controversy?, Hydrobiologia, 710, 3–21, https://doi.org/10.1007/s10750-012-1033-0, 2013.
Negandhi, K., Laurion, I., and Lovejoy, C.: Temperature effects on net greenhouse gas production and bacterial communities in arctic thaw ponds, FEMS Microbiol. Ecol., 92, fiw202, https://doi.org/10.1093/femsec/fiw202, 2016.
Ockenden, M. C., Hollaway, M. J., Beven, K. J., Collins, A. L., Evans, R., Falloon, P. D., Forber, K. J., Hiscock, K. M., Kahana, R., Macleod, C. J. A., and Tych, W.: Major agricultural changes required to mitigate phosphorus losses under climate change, Nat. Commun., 8, 161, https://doi.org/10.1038/s41467-017-00232-0, 2017.
Olefeldt, D., Heffernan, L., Jones, M. C., Sannel, B. K., Treat, C. C., and Turetsky, M. R.: Permafrost Thaw in Northern Peatlands: Rapid Changes in Ecosystem and Landscape Functions, edited by: Canadell, J. G. and Jackson, R. B., Ecosystem Collapse and Climate Change, Springer, 27–67, https://doi.org/10.4319/lo.2010.55.1.0115, 2021.
Opdal, A. F., Andersen, T., Hessen, D. O., et al.: Tracking freshwater browning and coastal water darkening from boreal forests to the Arctic Ocean, Limnol. Oceanogr. Lett., 8, 611–619, https://doi.org/10.1002/lol2.10320, 2023.
Oppenheimer, M., Glavovic, B. C., Hinkel, J. R., et al. (Eds.): IPCC Special report, Chap. 4, Sea Level Rise and Implications for Low-Lying Islands, Coasts and Communities, Cambridge University Press, Cambridge, UK and New York, NY, USA, 321–445, https://doi.org/10.1017/9781009157964.006.
Paerl, H. W. and Huisman, J.: Blooms Like It Hot, Science, 320, 57–58, https://doi.org/10.1126/science.1155398, 2008.
Paerl, H. W., Scott, J. T., McCarthy, M. J., et al.: It takes two to tango: when and where dual nutrient (N and P) reductions are needed to protect lakes and downstream ecosystems, Environ. Sci. Technol., 50, 10805–10813, https://doi.org/10.1021/acs.est.6b02575, 2016.
Paranaíba, J. R., Aben, R., Barros, N. G., et al.: Cross-continental importance of CH4 emissions from dry inland-waters, Sci. Total Environ., 814, 151925, https://doi.org/10.1016/j.scitotenv.2021.151925, 2021.
Piao, S., Friedlingstein, P., Ciais, P., et al.: Effect of climate and CO2 changes on the greening of the Northern Hemisphere over the past two decades, Geophys. Res. Lett., 33, 22, https://doi.org/10.1029/2006GL028205, 2006.
Rahel, F. J. and Olden, J. D.: Assessing the effects of climate change on aquatic invasive species, Conserv. Biol., 22, 521–533, https://doi.org/10.1111/j.1523-1739.2008.00950.x, 2008.
Raymond, P., Hartmann, J., Lauerwald, R., et al.: Global carbon dioxide emissions from inland waters, Nature, 503, 355–359, https://doi.org/10.1038/nature12760, 2013.
Reynolds, S. A. and Aldridge, D. C.: Global impacts of invasive species on the tipping points of shallow lakes, Glob. Change Biol., 27, 6129–6138, https://doi.org/10.1111/gcb.15893, 2021.
Ricciardi, A. and MacIsaac, H. J.: Recent mass invasion of the North American Great Lakes by Ponto–Caspian species, Trends Ecol. Evol., 15, 62–65, https://doi.org/10.1016/S0169-5347(99)01745-0, 2000.
Richardson, D. C., Holgerson, M. A., Farragher, M. J., et al.: A functional definition to distinguish ponds from lakes and wetlands, Sci. Rep., 12, 10472, https://doi.org/10.1038/s41598-022-14569-0, 2022.
Rockström, J., Steffen, W., Noone, K., et al.: A safe operating space for humanity, Nature, 461, 472–475, https://doi.org/10.1038/461472a, 2009.
Rockström, J., Gupta, J., Qin, D., et al.: Safe and just Earth system boundaries, Nature, 619, 102–111, https://doi.org/10.1038/s41586-023-06083-8, 2023.
Rosén, P.: Total organic carbon (TOC) of lake water during the Holocene inferred from lake sediments and near-infrared spectroscopy (NIRS) in eight lakes from northern Sweden, Biogeochemistry, 76, 503–516, https://doi.org/10.1007/s10533-005-8829-1, 2005.
Rühland, K. M., Smol, J. P., Wang, X., and Muir, D. C. G.: Limnological characteristics of 56 lakes in the Central Canadian Arctic treeline region, J. Limnol., 62, 9–27, 2003.
Rühland, K. M., Paterson, A. M., and Smol, J. P.: Hemispheric-scale patterns of climate-related shifts in planktonic diatoms from North American and European lakes, Glob. Change Biol., 14, 2740–2754, https://doi.org/10.1111/j.1365-2486.2008.01670.x, 2008.
Scheffer, M., Hosper, S. H., Meijer, M.-L., et al.: Alternative equilibria in shallow lakes, Trends Ecol. Evol., 8, 275–279, https://doi.org/10.1016/0169-5347(93)90254-M, 1993.
Scheffer, M., Carpenter, S., J., Foley, A. Folke, C., and Walker, B.: Catastrophic shifts in ecosystems, Nature, 413, 591–596, https://doi.org/10.1038/35098000, 2021.
Scheffer, M. and van Nes, E. H.: Shallow lakes theory revisited: various alternative regimes driven by climate, nutrients, depth and lake size, Hydrobiologia, 584, 455–466, https://doi.org/10.1007/s10750-007-0616-7, 2007.
Schulte-Uebbing, L. F., Beusen, A. H. W., Bouwman, A. F., and de Vries, W.: From planetary to regional boundaries for agricultural nitrogen pollution, Nature, 610, 507–512, https://doi.org/10.1038/s41586-022-05158-2, 2022.
Seekell, D. A., Pace, M. L., Heffernan, S. J., Holbrook, K., and Hambright, D. (Eds.): Special issue: Nonlinear dynamics, resilience and regime shifts in aquatic communities and ecosystems, Limnol. Oceanogr., 67, S1–S4, https://doi.org/10.1002/lno.12072, 2022.
Sereda, J., Bogard, M., Hudson, J., Helps, D., and Dessouki, T.: Climate warming and the onset of salinization: Rapid changes in the limnology of two northern plains lakes, Limnologica, 41, 1–9, https://doi.org/10.1016/j.limno.2010.03.002, 2011.
Sim, L. L., Chambers, J. M., and Davis, J. A.: Ecological regime shifts in salinised wetland systems, I. Salinity thresholds for the loss of submerged macrophytes, Hydrobiologia, 573, 89–107, https://doi.org/10.1007/s10750-006-0267-0, 2006.
Skerlep, M., Steiner, E., Axelsson, A. L., and Kritzberg, E. S.: Afforestation driving long-term surface water browning, Glob. Change Biol., 26, 1390–1399, 2020.
Smith, L. C., Sheng, Y., MacDonald, G. M., and Hinzman, L. D.: Disappearing Arctic Lakes, Science, 308, 1429, https://doi.org/10.1126/science.1108142, 2005.
Smith, V. H. and Schindler, D. W.: Eutrophication science: where do we go from here?, Trends Ecol. Evol., 24, 201–207, https://doi.org/10.1016/j.tree.2008.11.009, 2009.
Smol, J. P.: Lakes in the Anthropocene: Reflections on tracking ecosystem change in the Arctic, Excellence in Ecology Book Series, International Ecology Institute (ECI), Oldendorf/Luhe, Germany, ISBN: 9783946729303, 2023.
Smol, J. P. and Douglas, M. S. V.: Crossing the final ecological threshold in high Arctic ponds, P. Natl. Acad. Sci. USA, 104, 12395–12397, https://doi.org/10.1073/pnas.0702777104, 2007.
Smol, J. P., Wolfe, A. P., Birks, H. J. B., et al.: Climate-driven regime shifts in the biological communities of arctic lakes, P. Natl. Acad. Sci. USA, 102, 4397–4402, https://doi.org/10.1073/pnas.0500245102, 2005.
Sondergaard, M., Jensen, P. J., and Jeppesen, E.: Retention and internal loading of phosphorus in shallow, eutrophic lakes, Sci. World J., 1, 427–442, https://doi.org/10.1100/tsw.2001.72, 2001.
Spears, B. M., Futter, M. N., Jeppesen, E., et al.: Ecological resilience in lakes and the conjunction fallacy, Nat. Ecol. Evol., 1, 1616–1624, https://doi.org/10.1038/s41559-017-0333-1, 2017.
Spears, B. and Steinman, A. (Eds.): Internal Phosphorus Loading in Lakes: Causes, Case Studies, and Management, J. Ross Publish., ISBN: 978-1-60427-144-7, 2020.
Szklarek, S., Górecka, A., and Wojtal-Frankiewicz, A.: The effects of road salt on freshwater ecosystems and solutions for mitigating chloride pollution – A review, Sci. Total Environ., 805, 150289, https://doi.org/10.1016/j.scitotenv.2021.150289, 2022.
Stone, J. R., Saros, J. E., and Pederson, G. T.: Coherent late-Holocene climate-driven shifts in the structure of three Rocky Mountain lakes, Sage J., 26, 1103–1111, https://doi.org/10.1177/0959683616632886, 2017.
Tátrai, I., Boros, G., Gyögy, Á. I., et al.: Abrupt shift from clear to turbid state in a shallow eutrophic, biomanipulated lake, Hydrobiologia, 620, 149–161, https://doi.org/10.1007/s10750-008-9625-4, 2008.
Terhaar, J., Lauerwald, R., Regnier, P., Gruber, N., and Bopp, L.: Around one third of current Arctic Ocean primary production sustained by rivers and coastal erosion, Nat. Commun., 12, 169, https://doi.org/10.1038/s41467-020-20470-z, 2021.
Thrane, J. E., Hessen, D. O., and Andersen, T.: The absorption of light in lakes: Negative impact of dissolved organic carbon on primary productivity, Ecosystems, 17, 1040–1052, https://doi.org/10.1007/s10021-014-9776-2, 2014.
Tranvik, L., Downing, J. A., Cotner, J. B., et al.: Lakes and reservoirs as regulators of carbon cycling and climate, Limnol. Oceanogr., 54, 2298–2314, https://doi.org/10.4319/lo.2009.54.6_part_2.2298, 2009.
Turetsky, M. R., Abbott, B. W., Jones, M. C., et al.: Carbon release through abrupt permafrost thaw, Nat. Geosci., 13, 138–143, https://doi.org/10.1038/s41561-019-0526-0, 2020.
Valiente, N. P., Eiler, A., Allesson, L., et al.: Catchment properties as predictors of greenhouse gas concentrations across a gradient of boreal lakes, Front. Environm. Sci., 10, 1–16, https://doi.org/10.3389/fenvs.2022.880619, 2022.
van Dijk, G., Lamers, L. P. M., Loeb, R., Westendorp, P.-J., Kuiperij, R., van Kleef, H. H., Klinge, M., and Smolders, A. J. P.: Salinization lowers nutrient availability in formerly brackish freshwater wetlands; unexpected results from a long-term field experiment, Biogeochemistry, 143, 67–83, https://doi.org/10.1007/s10533-019-00549-6, 2019.
van Nes, E. H., Staal, A., van der Bolt, B., Flores, B. M., Batiany, S., and Scheffer, M. L.: What do you mean, “Tipping Point”, Trends Ecol. Evol., 31, 902–904, https://doi.org/10.1016/j.tree.2016.09.011, 2016.
Velthuis, M. and A. J.: Veraart Temperature Sensitivity of Freshwater Denitrification and N2O Emission – A Meta-Analysis, Global Biogeochem. Cy., 36, e2022GB007339, https://doi.org/10.1029/2022GB007339, 2022.
Wang, Y.-R., Hessen, D. O., Samset, B. H., and Stordal, F.: Evaluating global and regional land warming trends in the past decades with both MODIS and ERA5-Land land surface temperature data, Remote Sens. Environ., 280, 113181, https://doi.org/10.1016/j.rse.2022.113181, 2022.
Walseng, B., Jensen, T., Dimante-Deimantovica, I., et al.: Freshwater diversity in Svalbard: providing baseline data for ecosystems in change, Polar Biol., 41, 1995–2005, 2018.
Webb, E. E., Liljedahl, A. K., Cordeiro, J. A., et al.: Permafrost thaw drives surface water decline across lake-rich regions of the Arctic, Nat. Clim. Change, 12, 841–846, https://doi.org/10.1038/s41558-022-01455-w, 2022.
Wei, J., Fontane, L., Valiente, N., Dörsch, P., Hessen, D. O., and Eiler, A.: Trajectories of freshwater microbial genomics and greenhouse gas saturation upon glacial retreat, Nat. Commun., 14, 3234, https://doi.org/10.1038/s41467-023-38806-w, 2023.
Weyhenmeyer, G. A., Jeppesen, E., Adrian, R., et al.: Nitrate-depleted conditions on the increase in shallow northern European lake, Limnol. Oceanogr., 52, 1346–1352, https://doi.org/10.4319/lo.2007.52.4.1346, 2007.
Wik, M., Varner, R., Anthony, K., et al.: Climate-sensitive northern lakes and ponds are critical components of methane release, Nat. Geosci., 9, 99–105, https://doi.org/10.1038/ngeo2578, 2016.
Willcock, S., Cooper, G. S., Addy, J., and Dearing, J. A.: Earlier collapse of Anthropocene ecosystems driven by multiple faster and noisier drivers, Nat. Sustain., 6, 1331–1342, https://doi.org/10.1038/s41893-023-01157-x, 2023.
Williams, W. D.: Salinisation: A major threat to water resources in the arid and semi-arid regions of the world, Lakes Reserv. Res. Manag., 4, 85–91, https://doi.org/10.1046/j.1440-1770.1999.00089.x, 1999.
Williamson, C., Overholt, E., Pilla, R., et al.: Ecological consequences of long-term browning in lakes, Sci. Rep. 5, 18666, https://doi.org/10.1038/srep18666, 2016.
Woolway, R. I., Kraemer, B. M., Lenters, J. D., et al.: Global lake responses to climate change, Nat Rev. Earth Environ., 1, 388–403, https://doi.org/10.1038/s43017-020-0067-5, 2020.
Woolway, R. I., Sharma, S., and Smol, J.: Lakes in hot water: the impacts of a changing climate on aquatic ecosystems, BioScience, 72, 1050–1061, https://doi.org/10.1093/biosci/biac052, 2022.
Yan, X., Xu, X., Wang, M., et al.: Climate warming and cyanobacteria blooms: Looks at their relationships from a new perspective, Water Res., 125, 449–457, https://doi.org/10.1016/j.watres.2017.09.008, 2017.
Yang, H., Andersen, T., Dörch, P., Tominaga, K., Thrane, J. T., and Hessen, D. O.: Greenhouse gas metabolism in Nordic boreal lakes, Biogeochemistry, 126, 211–225, https://doi.org/10.1007/s10533-015-0154-8, 2015.
Yao, F., Livneh, B., Rajagopalan, B., Wang, J., Crétaux, J. F., Wada, Y., and Berge-Nguyen, M.: Satellites reveal widespread decline in global lake water storage, Science, 380, 743–749, https://doi.org/10.1126/science.abo2812, 2023.
Zhang, X., Ward, B. B., and Sigman, D. M.: Global Nitrogen Cycle: Critical Enzymes, Organisms, and Processes for Nitrogen Budgets and Dynamics, Chem. Rev., 120, 5308–5351, https://doi.org/10.1021/acs.chemrev.9b00613, 2020.
Zhu, Y., Purdy, K. J., Eyice, Ö., et al.: Disproportionate increase in freshwater methane emissions induced by experimental warming, Nat. Clim. Change, 10, 685–690, https://doi.org/10.1038/s41558-020-0824-y, 2020.