the Creative Commons Attribution 4.0 License.
the Creative Commons Attribution 4.0 License.
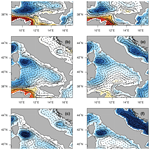
Climate change signal in the ocean circulation of the Tyrrhenian Sea
Iván M. Parras-Berrocal
Alfredo Izquierdo
Dmitry V. Sein
William Cabos
The Tyrrhenian Sea plays an important role in the winter deep water formation in the northwestern Mediterranean through the water that enters the Ligurian Sea via the Corsica Channel. Therefore, the study of the impact of the changes on the future climate on the Tyrrhenian circulation and its consequences represents an important issue. Furthermore, the seasonally dependent Tyrrhenian circulation, which is rich in dynamical mesoscale structures, is dominated by the interplay of local climate and the basin-wide Mediterranean circulation via the water transport across its major straits, and an adequate representation of its features represents an important modeling challenge. In this work we examine with a regionally coupled atmosphere–ocean model the changes in the Tyrrhenian circulation by the end of the 21st century under the RCP8.5 emission scenario, their driving mechanisms, and their possible impact on winter convection in the NW Mediterranean. Our model successfully reproduces the main features of the Mediterranean Sea and Tyrrhenian Basin present-day circulation. We find that toward the end of the century the winter cyclonic along-slope stream around the Tyrrhenian Basin becomes weaker. This weakening increases the wind work transferred to the mesoscale structures, which become more intense than at present in winter and summer. We also find that, in the future, the northward water transport across the Corsica Channel towards the Liguro-Provençal basin becomes smaller than today. Also, water that flows through this channel presents a stronger stratification because of a generalized warming with a freshening of upper and a saltening of intermediate waters. Both factors may contribute to the interruption of deep water formation in the Gulf of Lions by the end of the century.
- Article
(10706 KB) - Full-text XML
-
Supplement
(846 KB) - BibTeX
- EndNote
The Mediterranean Sea is a semi-enclosed basin with only one connection to the open ocean via the narrow and shallow Strait of Gibraltar (∼14 km width, ∼300 m depth). It is connected to the Black Sea through the Marmara–Bosphorus system and is divided into the eastern and western Mediterranean by the Strait of Sicily. Despite its small size, the Mediterranean Sea presents a thermohaline circulation which is driven by negative surface heat and freshwater budgets; i.e., it experiences a net heat and water loss to the atmosphere (e.g., Jordà et al., 2017), with deep and intermediate-depth open convection at specific sites in response to winter cooling (Bergamasco and Malanotte-Rizzoli, 2010). The surface component of the thermohaline circulation, the Atlantic Waters (AWs) flow into the Mediterranean Sea through the Strait of Gibraltar and then reach the eastern Mediterranean via the Sicily Strait. On its way to the east, the AWs experience a surface buoyancy loss due to evaporation. In the Levantine basin, water and heat loss due to air–sea fluxes drives intermediate-depth convection (down to 150–350 m) forming the so-called Levantine Intermediate Water, LIW (Wüst, 1961; Lascaratos et al., 1993; LIWEX group, 2003). This salty water mass, the LIW, flows to the west and then to the Atlantic Ocean, along with other Mediterranean intermediate and deep waters (Millot, 2009, 2019), through the deepest portion of the Strait of Gibraltar. In turn, the increased salt content of the LIW preconditions surface waters for deep water formation in winter in locations such as the Gulf of Lions, the Adriatic or the Aegean (MEDOC Group, 1970).
In the western Mediterranean, the Tyrrhenian Sea is well known for its complex configuration and bathymetry. Furthermore, its basin-scale circulation presents a strong seasonal variability and a very active, also seasonally dependent, mesoscale activity which are set by the interaction of the water exchange through the Strait of Sicily, the Corsica Channel and the Strait of Sardinia (see their location in Fig. 1), as well as by local atmospheric fluxes (e.g., Krivosheya and Ovchinnikov, 1973; Korres et al., 2000). The Tyrrhenian water column is composed of Modified Atlantic Water (MAW) at the surface, LIW at intermediate depths and Tyrrhenian Deep Water (TDW) at deeper levels (Astraldi et al., 2002; Vetrano et al., 2004). Observations and modeling works indicate a cyclonic winter circulation occupying the whole basin starting from a stream of MAW that enters the Tyrrhenian through the Sardinia Strait (Krivosheya and Ovchinnikov, 1973; Artale et al., 1994; Zavatarelli and Mellor, 1995), which is interrupted in summer (Artale et al., 1994; Gasparini et al., 2008). Below MAW, the LIW crosses the Sicily Strait and gets into the Tyrrhenian Sea, where flows are affected by the cyclonic circulation. At the Corsica channel, part of LIW deflects southward along the eastern coast of Corsica and Sardinia, while the rest continues flowing northward to the northwestern Mediterranean forming the so-called Ligurian Current (Sciascia et al., 2019). Thus, the outflow of LIW through the Corsica Channel to the Liguro-Provençal region is important as it may directly impact the so-called preconditioning phase (MEDOC Group, 1970) of deep water formation in the Gulf of Lions (Schroeder et al., 2010). Regarding the mesoscale circulation, permanent and recurrent structures have been spotted over recent years thanks to the use of satellite images and high-resolution ocean circulation models (Béranger et al., 2004; Vetrano et al., 2010; Iacono et al., 2013; Napolitano et al., 2016; de la Vara et al., 2019). In particular, mesoscale circulation has been found to be especially active in summer.
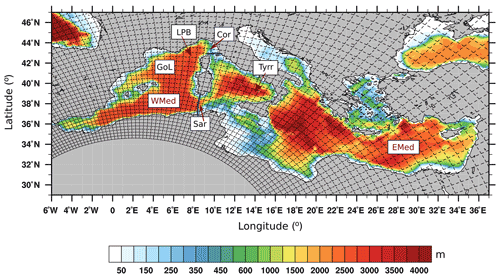
Figure 1Bathymetry of the Mediterranean Sea adopted in ROM, in m, together with the oceanic grid (only one out of four lines are drawn). “WMed” and “EMed” refer to the western and eastern Mediterranean Sea, respectively. The arrows next to “Sar” and “Cor” indicate the location of the transects in which the transport across the Sardinia Strait and the Corsica Channel are measured (their coordinates are indicated in Figs. 4 and 5). “Tyrr” stands for Tyrrhenian, “GoL” for Gulf of Lions and “LPB” to Liguro-Provençal basin.
In the current context of climate change, the Mediterranean Sea has been identified as one of the regions in which the warming signal is amplified. Thus, this sea is widely considered as a climate change “hot-spot” for the scientific community (Giorgi, 2006). This projected warming may have an impact on the air–sea fluxes, deep water convection (Parras-Berrocal et al., 2020, 2021) and the overall Mediterranean Sea circulation (Soto-Navarro et al., 2020). As stressed above, the Tyrrhenian Sea shows seasonal variability and features recurrent structures that are heavily dependent on the large-scale Mediterranean circulation and the local air–sea fluxes. Therefore, the impact that the changes induced by the future warming in the ocean and the atmosphere may have on the circulation in that region is of particular interest. Also, waters that exit the Tyrrhenian via the Corsica Channel have an impact on the hydrology of the Liguro-Provençal area and thus on the deep water formation in the Gulf of Lions (Astraldi et al., 1999). Thus, the modeling challenge that the representation of the complex circulation patterns and the important role that Tyrrhenian waters play in the preconditioning for deep water formation in the Gulf of Lions make the study of the evolution of the Tyrrhenian Sea circulation under climate change an interesting topic of research. Changes in the water properties within the Tyrrhenian Sea inherent to climate change may have an impact on deep winter convection in the Gulf of Lions, which is important for the hydrographic properties of the Mediterranean Sea water, its thermohaline circulation and thus sea-bottom ventilation. Furthermore, to our knowledge, there are no published papers devoted to the study of changes in the Tyrrhenian surface circulation in the future climate. As climate change simulations with state-of-the-art global models do not have the necessary horizontal resolution, the best tool available for the study of changes in the regional ocean circulation and the corresponding driving mechanisms are the atmosphere–ocean regionally coupled models (AORCMs; e.g., Rummukainen, 2016). Only the oceanic and atmospheric components of regional models can reach the necessary horizontal resolution to simultaneously capture basin-scale and mesoscale processes in this region (e.g., Somot et al., 2006). The main aim of this work is to study the impact of climate change on the variability of the surface circulation of the Tyrrhenian Sea by the end of the century, as well as to identify the implied driving mechanisms and to examine the possible impact of these changes on winter convection in the NW Mediterranean Sea. To that end we use the regionally coupled climate model ROM (Sein et al., 2015), which has been extensively adopted to study aspects related to the climate of the Mediterranean Sea (e.g., Darmaraki et al., 2019; Parras-Berrocal et al., 2020). A great advantage of the ROM configuration used in this study is that it represents an open Strait of Gibraltar (see Fig. 1) allowing the propagation of Atlantic Ocean climate change signals into the Mediterranean Sea (Parras-Berrocal et al., 2020, 2021). This is of utmost importance as the surface freshening of the eastern North Atlantic, which is a robust feature within CMIP5 RCP8.5 projections (Levang and Schmidt, 2020; Soto-Navarro et al., 2020), may have profound impacts on the Mediterranean Sea (Parras-Berrocal et al., 2020, 2021) in general and on the Tyrrhenian Sea in particular. This article is structured as follows. In Sect. 2, the model setup is described. In Sect. 3, the present-day and future geostrophic circulation of the Tyrrhenian Basin and changes in the transport across the Sardinia Strait and the Corsica Channel are studied. In Sect. 4, the causes and the possible consequences of the reported changes are analyzed. The conclusions are offered in Sect. 5.
Here we use the regionally coupled climate model ROM (REMO-OASIS-MPIOM; Sein et al., 2015) comprising the atmospheric component REMO (REgional MOdel; Jacob and Podzun, 1997; Jacob, 2001), the oceanic component MPIOM (Max Planck Institute Ocean Model; Maier-Reimer, 1997; Marsland et al., 2003; Jungclaus et al., 2013) and the OASIS coupler (Valcke et al., 2003). REMO (Déqué et al., 2012; Jimenez-Guerrero et al., 2013; Kotlarski et al., 2014) and ROM (Sein et al., 2014; Cabos et al., 2018; Darmaraki et al., 2019) have been successfully applied to study the regional climate and climate change signal in different regions of the world, with a performance comparable to the best state-of-the-art models (Cabos et al., 2017; Sein et al., 2020).
MPIOM is a well-established global ocean model that has been extensively used not only for global climate studies as part of the different versions of MPI-ESM (Max-Planck-Institut für Meteorologie Earth System Model; Giorgetta et al., 2013), but also for regional studies (e.g., Mathis et al., 2018; Izquierdo and Mikolajewicz, 2019; Liu et al., 2021). MPIOM has an orthogonal curvilinear grid that, in this configuration, achieves a maximum horizontal resolution of about 7 km (mesoscale eddy-rich) near the Gibraltar Strait, decreasing down to 25 km at the eastern coast of the Mediterranean. On the vertical, MPIOM counts with 40 unevenly spaced z levels with a variable thickness that ranges from 16 at the surface to 650 m near the seafloor. Unlike most of the regional models devoted to the study of the Mediterranean Sea, the water exchange at Gibraltar and the Dardanelles in ROM is not parameterized, and Atlantic water properties are not relaxed towards climatological values in the areas adjacent to the straits. Actually, the oceanic component of our coupled model is global, and the boundaries of the atmospheric component are located far from Gibraltar. As the atmospheric domain is large and encompasses most of the North Atlantic, MPI-ESM influences the oceanic properties through the large-scale forcing on the atmospheric component of ROM; in turn the large-scale North Atlantic Ocean climate change signal can propagate into the Mediterranean through the open Strait of Gibraltar. This is relevant as it allows the surface freshening signal projected by the driving MPI-ESM in the eastern North Atlantic at the end of the 21st century to be accounted for (Parras-Berrocal et al., 2020).
In REMO a rotated grid with the center of the model domain located around the Equator is used. It has a constant horizontal resolution of 0.25∘ and a total of 27 hybrid levels. The atmospheric domain includes both the North Atlantic and the Mediterranean Sea (see Fig. S1 in the Supplement). The global Hydrological Discharge Model (HD; Hagemann and Dümenil Gates, 2001) computes river runoff at 0.5∘ resolution and is coupled to both the ocean and the atmosphere.
OASIS (Valcke et al., 2003) is used to couple REMO and MPIOM, and atmospheric and oceanic fields are exchanged with a 3 h coupling time step (see Sein et al., 2015). For more details on the ROM setup we refer the reader to Cabos et al. (2020). ROM has been shown to be able to provide a regional signal that is significantly different from the one provided by the driving model (Limareva et al., 2017; Cabos et al., 2018; Sein et al., 2020).
To study the impact of climate change on the mesoscale circulation of the Tyrrhenian, we use a simulation in which ROM is forced by the low-resolution version of MPI-ESM under the high-emission RCP8.5 CMIP5 scenario (Taylor et al., 2012), which is expected to have the strongest impact on the Tyrrhenian circulation. ROM and MPI-ESM share the same oceanic component, but the MPIOM setup used in MPI-ESM differs from the setup used in ROM. In particular, MPI-ESM has the higher horizontal resolution in the North Atlantic deep water formation areas, with a minimum grid spacing of about 15 km around Greenland (Jungclaus et al., 2006). In ROM, the higher-resolution areas are located near the North American and North African coasts, having the highest resolution in the southern western Mediterranean and northwestern Africa, reaching 7 km in the Gibraltar Strait and the region of the Alboran Sea. Also, ROM has a good representation of the diurnal cycle (3 h coupled time step), which is neglected in the MPI-ESM simulations. The climate change simulations start from the last state of the present-day simulations and cover the 2006–2099 time period. This ROM configuration has been extensively validated in Parras-berrocal et al. (2020), but to ease the reading we provide in the Supplement the validation of seasonal and interannual sea-surface salinity (SSS) variability.
In this section we focus on the geostrophic circulation in order to use available gridded products derived from satellite altimetry for validation. For estimating ROM present-day climate we use the last 30 years of the historical run, namely from 1976 to 2005, which is widely used as a reference period for present climate, then we assess circulation changes by comparing this reference period with the future one (2070–2099). However, for validation purposes, first we show the present-day (1993–2005) Mediterranean-scale geostrophic circulation simulated by ROM, as this period is coincidental with the AVISO satellite altimetry gridded product. Then, we concentrate on the validation of the present-day geostrophic circulation of the Tyrrhenian Sea and study how its basin-scale and mesoscale-size circulation changes by the end of the century (2070–2099). Additionally, we also examine present-day and future vertical sections of velocity, salinity and temperature at the Sardinia Strait and the Corsica Channel.
3.1 Present-day Mediterranean geostrophic circulation
Here we study the ability of ROM to represent the seasonal, present-day geostrophic circulation of the Mediterranean Sea in comparison to high-resolution altimeter data from the Mediterranean version of AVISO (Copernicus Marine and Environment Monitoring Service; https://doi.org/10.48670/moi-00141). AVISO provides multimission altimeter gridded sea-surface heights and derived variables over the Mediterranean Sea with a horizontal resolution of . We remark that the time period chosen for this validation is made to match with AVISO data availability (1993–2005). The reason we focus on the surface geostrophic circulation is twofold. On the one hand, a complete validation of the ability of ROM to reproduce the main features of the Mediterranean Sea characteristics has already been done in, for example, Parras-Berrocal et al. (2020) and Soto-Navarro et al. (2020). On the other hand, an adequate representation of the main features of the present-day circulation is required in order to study the future circulation and determine the causal mechanisms responsible for the changes in the geostrophic circulation by the end of the 21st century.
In winter ROM captures the main features of the Mediterranean geostrophic circulation inferred from AVISO (Fig. 2a and c), that is, AWs entering the Mediterranean Sea at the surface and the development of the so-called Alboran anticyclonic gyres. AWs keep on flowing along the African coast on their way to the east forming the Algerian current, characterized by a large amount of mesoscale activity (Millot, 1985). Part of these AWs, modified in the Algerian basin, flows north to the Ligurian and Provençal areas and enters the Tyrrhenian Sea, and the remaining waters go through the Sicily Strait to the eastern Mediterranean (Ovchinnikov, 1966). Then, MAW continues to flow along-slope to complete a cyclonic gyre around the Mediterranean Sea. We also observe several areas characterized by a cyclonic circulation and large negative values of sea-surface height (SSH) associated with the sinking of surface waters due to convection (i.e., Gulf of Lions, Adriatic Sea, Aegean Sea and Levantine basin). The mesoscale-size activity is smaller in ROM than in AVISO due to the lower horizontal resolution of the model in comparison to satellite data. This is more evident in the eastern basin, as MPIOM horizontal resolution there is lower (see Fig. 1).
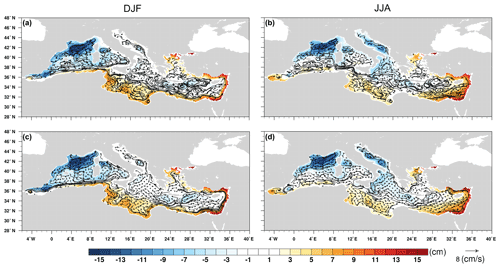
Figure 2Winter (a, c) and summer (b, d) averages of present-day geostrophic circulation (vectors, cm s−1) and sea-surface height (SSH, colors, cm) from AVISO (a, b) and ROM (c, d). For comparison we use the 1993–2005 period, when both model and observed data are available.
In summer, ROM also reproduces well the main characteristics of the Mediterranean circulation from AVISO (Fig. 2b and d). First, ROM shows the slowdown in the geostrophic velocities along the African coast. Also, the summer intensification of the Alboran Gyres is properly captured (Vargas-Yáñez et al., 2002; Dastis et al., 2018). The four previously mentioned cyclonic gyres where winter convection occurs are still distinguished in summer. In this season, the along-slope, cyclonic gyre around the Mediterranean remains, but most of the MAW flows via the Sicily Strait with little inflow into the Tyrrhenian Sea. As in winter, in summer, ROM ocean resolution shows up in the mesoscale representation: mesoscale-size structures are properly reproduced in the western basin and in the Adriatic Sea, and not so well in the eastern basin.
From a quantitative perspective, ROM provides quite a realistic representation of the basin-scale circulation and its seasonal variability. Major gyres are also well represented, but the reproduction of mesoscale-size structures is limited by ROM horizontal resolution, which is fair in the western basin (7 km in the Alboran Sea), but relatively low in the eastern basin (25 km) (see Fig. 1). Therefore, AVISO shows stronger mesoscale activity, larger spatial variability of the SSH and higher geostrophic velocities than ROM. Despite those differences, a good correlation is found between the SSH from ROM and AVISO in both seasons (0.73 for winter and 0.67 for summer). Thus, ROM resolution in the region allows for a realistic representation of the main features of the surface circulation, especially in the western Mediterranean (Parras-Berrocal et al., 2020). Overall, we can conclude that ROM is able to capture the main features of the Mediterranean circulation and its seasonal variability, and the results are comparable to those obtained with other well-established models (e.g., Bergamasco and Malanotte-Rizzoli, 2010).
3.2 Present-day and future geostrophic circulation in the Tyrrhenian Sea
In winter, in agreement with AVISO and previous modeling and observational works, the large-scale circulation pattern that arises from ROM points to a basin-wide, cyclonic stream around the Tyrrhenian Sea (Fig. 3a and b). MAW enters the Tyrrhenian through the southeastern part of the Sardinia Strait by the western tip of Sicily. Part of this flow completes the cyclonic, along-slope gyre, exiting via the northwestern portion of the Sardinia Strait, where a side-by-side counter-flow is observed, whilst part of it enters the Ligurian region via the Corsica Channel. Two prominent cyclonic, dynamical structures are overlapped to the basin-wide cyclonic gyre. The first one is the Bonifacio Gyre, which arises to the east of this narrow strait separating Corsica and Sardinia and is related to the wind funneling through the Strait of Bonifacio (Artale et al., 1994; Vigo et al., 2019). The second one is a recirculation structure off Sardinia at the southern boundary of the Tyrrhenian Sea. These structures have been identified as permanent or quasi-permanent features of the Tyrrhenian (Artale et al., 1994; Rio et al., 2007; Rinaldi et al., 2010; Vetrano et al., 2010; Iacono et al., 2013; Napolitano et al., 2016).
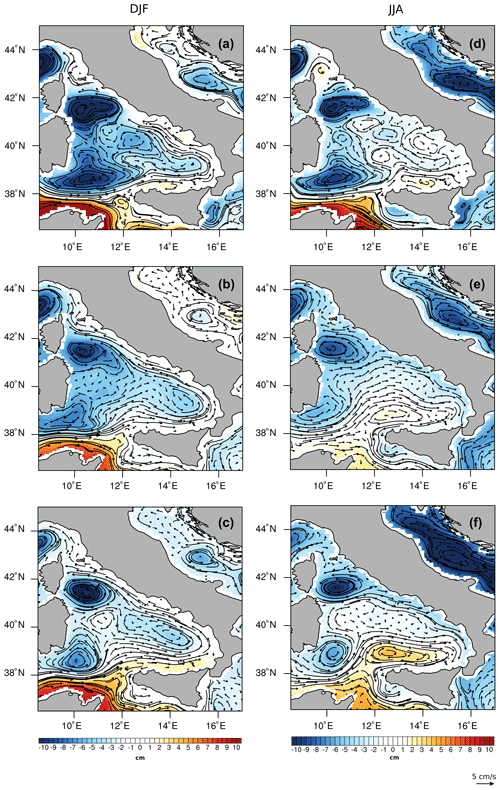
Figure 3Winter (a–c) and summer (d–f) averages of geostrophic circulation (arrows, cm s−1) and sea-surface height (SSH, colors, cm) computed (a, d; 1993–2005) from AVISO, (b, e) present-day reference period (1976–2005) and (c, f) future (2070–2099) with ROM.
In summer, the basin-scale circulation presents important differences with respect to the winter situation, and ROM is able to capture the main features of the typical summer pattern (Fig. 3d and e). For instance, the winter basin-wide cyclonic circulation around the Tyrrhenian does not arise. Whilst, as in winter, MAW enters the basin through the southern part of the Sardinia Strait, the development of several dynamical structures to the east of the basin reverses the circulation along the southern and eastern coasts of the Tyrrhenian, hampering the direct connection between the southern and northern openings. In general, more dynamical structures develop than in winter. The Bonifacio Gyre stretches zonally, and the water transport across the Corsica Channel becomes smaller (Astraldi and Gasparini, 1992). The recirculation structure near Sardinia becomes more defined. The anticyclonic circulation becomes predominant to the southeast of the Tyrrhenian, over the Sicilian coast (Iacono et al., 2013; Napolitano et al., 2016; de la Vara et al., 2019). In this season, ROM captures the most prominent dynamical structures depicted from AVISO data, but not all the secondary ones. This was also the case in previous works addressing the Tyrrhenian surface circulation (e.g., de la Vara et al., 2019). The differences between the representation of the dynamical structures in ROM and AVISO are, as explained above, due to the lower resolution of the ocean model relative to AVISO altimeter data. However, this should not be problematic given that the main aim of this work is to study the changes induced in the Tyrrhenian circulation in response to climate change.
In the projected scenario, the winter circulation patterns are qualitatively similar to those found in the present-day simulation (Fig. 3a–c). However, the Tyrrhenian Basin cyclonic circulation becomes weaker and the two previously mentioned dynamical mesoscale structures become larger and more intense, especially the Bonifacio Gyre. This may be related to the weakening of the basin-scale cyclonic stream, which allows for the intensification and zonal stretching of these structures. In other words, although the present-day winter circulation patterns are still visible, a trend towards the typical present-day summer geostrophic circulation is observed, i.e., a decrease in the intensity of the basin-scale current velocity pattern, larger and faster dynamical structures, and a reduced water transport to the Ligurian basin through the Corsica Channel. The future summer circulation closely resembles the current summer pattern (Fig. 3d–f). This notwithstanding, in line with what was found for winter, a clear intensification of the dynamical structures and of the reversed eastern boundary current is observed. The zonal stretching of the Bonifacio Gyre limits, even more than at present, the water transport across the Corsica Channel. Our results agree with those from Ser-Giacomi et al. (2020), in which the authors find an increase in the eddy kinetic energy (i.e., mesoscale activity) in the Tyrrhenian Sea by the end of the century. In general, we can thus conclude that, although the typical winter and summer patterns can be still recognized, a transition towards a summer pattern can be observed. These results are consistent with what can be expected in a warmer climate, and the implied causal mechanisms will be analyzed in detail in the Discussion.
3.3 Impact of climate change on the boundary conditions at the Tyrrhenian straits
Here we focus on the impact of climate change at the current velocity across the Sardinia Strait and the Corsica Channel. Figures 4 and 5 show vertical cross sections of current ocean velocity for the Sardinia Strait and the Corsica Channel, respectively, for present-day (a, d) and future (b, e) climate, as well as the differences between future and present values (c, f). Starting the analysis from the Sardinia Strait, it is important to recall that, at present, in winter, MAW enters the Tyrrhenian to the south of this strait, completes a cyclonic gyre around the basin and partially exits the Tyrrhenian via its northern portion (Fig. 3a–c). This explains the side-by-side inflow and outflow at shallow levels (Fig. 4a; see for example Vetrano et al., 2004; de la Vara et al., 2019). The MAW (σ= 27.00–28.90; Serravall and Cristofalo, 1999) jet stream flows into the Tyrrhenian from the surface to about 150–250 m depth with velocities between 0.10 and 0.15 m s−1, while it flows out with velocities close to 0.05 m s−1. Below MAW, LIW (σ= 28.85–29.10; Lascaratos et al., 1993; Hayes et al., 2019) flows out of the Tyrrhenian (negative values) with the largest speeds of around 0.05 m s−1 at 250–350 m depth. In the future climate there is a decrease in the water density, more remarkable at the surface, due to the impact of global warming in water mass characteristics. The surface velocities decrease, with a weakening of both the MAW inflow and outflow, but the LIW outflow to the north of the strait intensifies with speeds over 0.05 m s−1 (Fig. 4b and c). In other words, surface currents associated with MAW weaken, whilst intermediate-depth currents associated with LIW transport intensify. The weakening of the surface flow is associated with a flattening of the isopycnals and a remarkable lightening of surface waters, which leads to an increased stratification, in line with what happens in the Gulf of Lions region (Parras-Berrocal et al., 2021). In summer, as observed in Fig. 3, the surface circulation pattern is substantially different from that in winter, with a weaker and shallower MAW stream entering the Tyrrhenian through the center of the strait and outflow currents elsewhere in the studied depth range (Fig. 4d). Also, the water column in the first 200 m is more stratified and lighter than in winter due to summer insolation. In the future climate, current velocities experience a generalized decrease, but there is a notable intensification of velocities in the depth range and location of the present LIW, with a maximum from about 150 to 400 m depth by the Sardinia shelf slope (Fig. 4d and e). It should be noted that, regardless of the season, in the future climate water density decreases throughout the first 400 m (Fig. 4a–f). This is related to the generalized temperature increase in the Mediterranean and the freshening of the MAW (Parras-Berrocal et al., 2020).
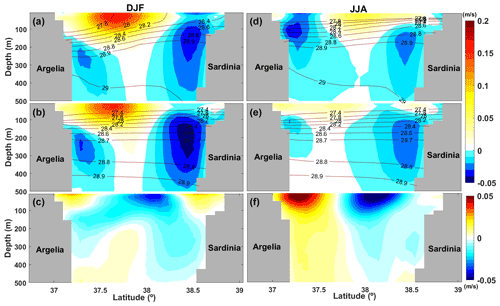
Figure 4Winter (a–c) and summer (d–f) vertical cross sections of current velocity across the Sardinia Strait (36.73∘ N, 8.04∘ E to 39.04∘ N, 8.79∘ E; see Fig. 1), in m s−1, for present (a, d) and future (b, e) climate as computed from ROM. Positive values indicate eastward flow and negative values the opposite. Isopycnals are also depicted. Differences between ocean current velocities in the future and present climate are shown in panels (c, f). Note that only the upper 500 m of the water column are shown for a more detailed visualization of surface velocities.
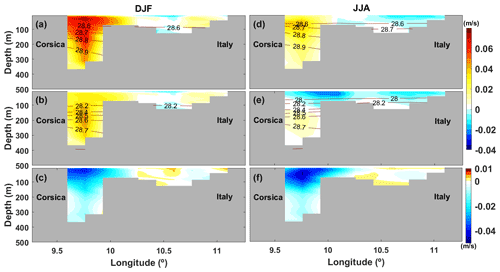
Figure 5Winter (a–c) and summer (d–f) vertical cross sections of current velocity across the Corsica Channel (42.53∘ N, 9.26∘ E to 42.80∘ N, 11.26∘ E; see Fig. 1), in m s−1, for present (a, d) and future (b, e) climate as computed from ROM. Positive values indicate northward flow and negative values the opposite. Isopycnals are also depicted. Differences between ocean current velocities in the future and present climate are presented in panels (c) and (f).
Present-day velocities in the Corsica Channel are positive (outflowing) all over the water column and feature a maximum over 0.06 m s−1 at the surface in winter (Fig. 5a). This implies that, as seen in Fig. 3a–c, part of the MAW that flows around the Tyrrhenian does not complete the cyclonic gyre around the basin and exits via the Corsica Channel. The core of this outflow is located at the west of the channel within the upper 30 m, and velocities decrease gradually with depth. In the future, the same pattern remains but velocities become weaker, and the maximum, which is close to 0.04 m s−1 (Fig. 5b and c), is not located at the surface but further down close to a depth of 200 m. This is consistent with the results found in the Sardinia Strait, i.e., the MAW stream that enters the Tyrrhenian weakens in the future. This is also in line with the weakening of the cyclonic stream around the Tyrrhenian Basin and the enhancement of dynamic structures with a shift towards the summer geostrophic circulation pattern. In summer, at present, as can be seen in Fig. 5d and in agreement with observations, northward current velocities attain a very small magnitude with values not larger than 0.04 m s−1, with maxima located at 70–100 m depth. In the future, positive (northward) velocities undergo a strong reduction, remaining positive at the depth of the channel, but negative values arise at shallow levels, with magnitudes larger than 0.02 m s−1 (Fig. 5d and e). Again, density experiences a future decrease all over the water column.
From these results we can conclude that, in the future climate, in winter, MAW enters the Tyrrhenian at a slower pace than at present. This is consistent with the slowdown of the along-slope, cyclonic stream that borders the Tyrrhenian in this season, as seen in Figs. 3a–c and 4a and b. This is also the case for the MAW entering the Liguro-Provençal basin via the Corsica Channel, as illustrated in Fig. 5a and b. This northward branch of the stream that flows close to the Corsica coast weakens significantly in the future.
We now examine the changes in the water properties across the Sardinia Strait and Corsica Channel. These are shown in Figs. 6–9, which are equivalent to Figs. 4 and 5 but constructed for temperature and salinity. We would like to emphasize that a detailed validation of ROM's capability to simulate the present-day salinity and temperature over the Mediterranean Sea has been extensively studied (we refer the reader to Darmaraki et al., 2019; Parras-Berrocal et al., 2020; and Soto-Navarro et al., 2020, for details). In particular, ROM improves a strong negative bias in SSS present in MPI-ESM. Presently, winter temperatures in the upper 200 m of the Sardinia Strait vary between 15.5 ∘C to the south to 14 ∘C to the north (Fig. 6a). This meridional temperature gradient relates to the fact that MAW enters the Tyrrhenian to the south and exits the basin to the north after completing a cyclonic gyre. Down from that depth, temperature is quite homogeneous and exhibits values close to about 14 ∘C. In summer, the water column is, as expected, stratified due to the development of the seasonal thermocline, but the meridional temperature gradient is less marked due to the decrease in the MAW inflow into the Tyrrhenian. Values are close to 20 ∘C at the surface and about 14 ∘C at a depth close to 100 m (Fig. 6d). From that depth downwards, temperature is close to 14 ∘C or less. In the future, the winter and summer vertical structure of the water column is qualitatively similar to that at present, but with warmer temperatures (Fig. 6b, c, e, and f). In winter, surface temperature ranges between 17.5 and 17 ∘C at the surface, and from 150 to 200 m depth water temperature is close to 16 ∘C. In summer, surface temperature is even warmer than 22 ∘C. From that depth downwards, temperature is about 16 ∘C.
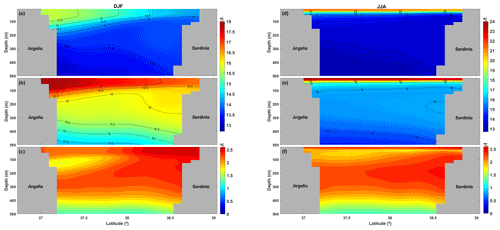
Figure 6Winter (a–c) and summer (d–f) vertical cross sections of temperature, in ∘C, through the Sardinia Strait for present (a, d) and future (b, e) climate as computed from ROM. Isotherms are also depicted. Differences between water temperature in the future and present climate are presented in panels (c) and (f).
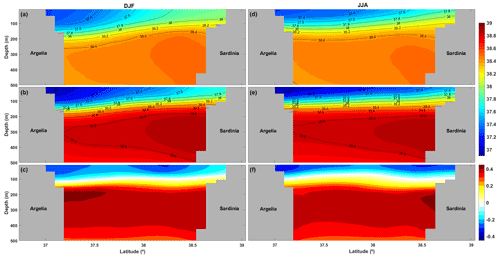
Figure 8Winter (a–c) and summer (d–f) vertical cross sections of salinity, in psu, through the Sardinia Strait for present (a, d) and future (b, e) climate as computed from ROM. Isohalines are shown. Differences between ocean salinity in the future and present climate are presented in panels (c) and (f).
At present, in the Corsica Channel, in winter, temperature is quite homogeneous and takes values close to 14 ∘C with values slightly higher at the surface (Fig. 7a). In the future, winter temperature is homogeneous as well but shows a nearly 2 ∘C increase all over the water column (Fig. 7b and c). In summer, the water column is thermally stratified with values close to 20 ∘C at the surface and near 14 ∘C below the thermocline down to the seafloor (Fig. 7d). In the future summer, the water column remains stratified but warmer, with values around 24 ∘C at the surface and close to 16 ∘C from about 100 m depth (Fig. 7d and e).
Focusing now on salinity we observe that, at present, in the Sardinia Strait, salinity is lower at the surface, with a marked north–south gradient and turning more homogeneous from about 250 m (Fig. 8a). Specifically, in winter, surface salinity is close to 37.5 psu to the south and closer to 38 psu to the north of the strait. These results are in agreement with those from Beranger et al. (2004) and de la Vara et al. (2019). This salinity gradient reflects a side-by-side channel flow due to the entrance of fresher MAW from the Algerian Current to the south of the Tyrrhenian which completes a cyclonic gyre around the basin and exits the Tyrrhenian to the north of the strait with a higher salinity. Further down, from about 250 m, salinity is close to 38.4 psu, which is typical for LIW (e.g., Astraldi et al., 2002). In the future, the winter salinity pattern is qualitatively similar to that at present, but values are different (Fig. 8b and c). On the one hand, MAW that enters and exits the Tyrrhenian is slightly fresher than today, which is related to the North Atlantic influence as previously reported by Parras-Berrocal et al. (2020) and Soto-Navaro et al. (2020), respectively. On the other hand, LIW is saltier than today, with a LIW core with a salinity close to 38.8 psu.
In summer, at present, the entrance of MAW to the Tyrrhenian via the Sardinia Strait is more limited, and the cyclonic stream around the basin is limited to the southern Tyrrhenian Sea, so that the north–south salinity gradient across the Sardinia Strait is more modest than in winter, and thus isohalines are less tilted, i.e., more horizontal (Fig. 8d). From about 150 m depth, salinity varies less and takes values close to 38.4 psu. In the future, MAW also becomes fresher than at present. LIW becomes, as in winter, saltier than today, with values close to 38.8 psu for the LIW core (Fig. 8e and f).
In the Corsica Channel, at present, in the winter situation, salinity is lowest at the surface and increases with depth (Fig. 9a). Its value is close to 38 psu at the surface (see for example Béranger et al., 2005; de la Vara et al., 2019) and close to 38.4 psu at the bottom of the channel, which are values close to those from Béranger et al. (2005). In the future, salinity stays similar or lower at the surface, but waters become saltier from about 100 m to the seafloor, reaching up to 38.8 psu (Fig. 9b and c). In summer, the situation is qualitatively like that reported for the winter season (Fig. 9d–f). Salinity changes due to the freshening of the Atlantic inflow is a robust feature which has been found in previous works (e.g., Parras-Berrocal et al., 2020, 2021; Soto-Navarro et al., 2020). In the latter paper the authors show that the freshening of the Atlantic waters leads to a decrease in the salinity of the inflow in Gibraltar, making this fresher Atlantic water the main contributor to the surface freshening in the western Mediterranean. This surface freshening will make a strong contribution to the reinforcement of the stratification of the waters that enter the Liguro-Provençal basin, as will be tackled in the Discussion.
At this point it is interesting to understand the causes and consequences of the projected changes in the Tyrrhenian Sea geostrophic circulation and water transport across its main straits.
4.1 Drivers for the future changes in the geostrophic circulation of the Tyrrhenian
Here we investigate the role of winds in the changes of the geostrophic circulation projected for the end of the century, as seen in the previous section. The surface wind pattern over the Tyrrhenian Sea presents a clear seasonality. We observe that in present-day winter the there is a predominance of westerly winds (Fig. 10a). The strongest wind speeds occur to the east side of Sardinia and Bonifacio straits, due to wind funneling (see for example Gérigny et al., 2015). These areas of enhanced wind speed coincide with those where the main dynamical structures are found (see Fig. 3a–c and de la Vara et al., 2019, for example). The lowest wind speeds occur near coastal areas, with values even below 1 m s−1. Whilst wind direction does not display important differences in the future, its module shows a general decrease in the future, but especially in the Sardinia and Bonifacio Straits, related mainly to a speed decrease, with a reduction of the largest values from 6 m s−1 to values between 4 and 5 m s−1 (Fig. 10c and e). The wind slackening found near the entrance of the Sardinia Strait may be an important trigger for the slowdown of the cyclonic stream around the basin which is fed by MAW.
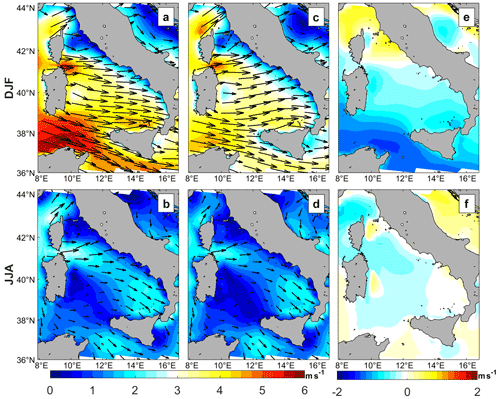
Figure 10Winter (a, c, e) and summer (b, d, f) averages of the wind speed module (colors, m s−1) and direction (arrows) for present (a, b) and future (c, d) climate as computed from ROM. Differences between the wind speed module in the future and present climate are shown in panels (e) and (f).
In summer, winds are far weaker than in winter, with an overall decrease in the magnitude of the westerlies (Fig. 10b). Once more, the greatest wind speeds arise to the east of the Bonifacio Strait, with values close to 2 m s−1. In this season, high wind speeds also occur to the southeast of the Tyrrhenian, where two important dynamical structures are found (see Fig. 3d and e). As found for the winter season, the future pattern of surface wind is qualitatively similar to the present one, but with remarkably lower wind speeds (Fig. 10d and f). The general decrease in the future surface wind speed is in line with results from Ser-Giacomi et al. (2020).
We now study the relationship between the wind representation and the ocean circulation. To that end, we compute the mean wind work in the Tyrrhenian (e.g., Renault et al., 2016). This is calculated using the following expression:
where ρo is the mean value of the sea water density assumed 1020 kg m−3, uo and vo are the zonal and meridional components of the ocean velocity of the five more superficial ocean model levels (down to 51 m) in m s−1, and τx and τy represent the zonal and meridional components of the wind stress, respectively, in N m−2. The overbars indicate climatological averages estimated for the present and future time periods. The wind work represents the exchange of kinetic energy between the atmosphere and the ocean. Positive values indicate that both winds and ocean currents move in the same direction; i.e., wind provides kinetic energy to the ocean. Negative values, however, indicate that they move in different directions and thus that winds reduce the ocean's kinetic energy. Positive and negative side-by-side values either correspond to water recirculation or the presence of an eddy. Overall, as shown in Fig. 11a and b, the winter and summer patterns found for present-day mean wind work in the Tyrrhenian are in line with those found in de la Vara et al. (2019). In winter, the combination of westward winds and a cyclonic stream around the Tyrrhenian leads to positive values from the Sardinia Strait to the easternmost part of the basin (Fig. 11a). Then, negative values arise as the water continues to flow to the northwest of the Tyrrhenian Sea. Side-by-side positive and negative values show up to the east of the Bonifacio Strait, where the Bonifacio Gyre is found. Negative values are also present in the recirculation area near the Sardinia Strait. In the future, qualitatively, the same basin-scale pattern remains, but positive and negative values of the wind work become remarkably higher in the Bonifacio Gyre with up to 8 cm3 s−3 in that region (Fig. 11c and e). The future enhancement of the wind work, especially in the areas where the dynamical structures appear, is thus not caused by wind speed intensification. The enhancement of the wind work may be related to the weakening of the cyclonic stream around the Tyrrhenian, which makes stronger the transference of kinetic energy from the wind to the Bonifacio Gyre and could be responsible for its intensification and increased extension. The strength of the Bonifacio Gyre is determined by the energy input by the wind stress and its interaction with the cyclonic jet, which employs part of the wind energy for the shedding of smaller-scale eddies, constraining the size of the gyre. In the future, the weakening of the stream makes it more stable, reducing the shedding, which is reflected by the increase in the size of the Bonifacio Gyre and its strengthening.
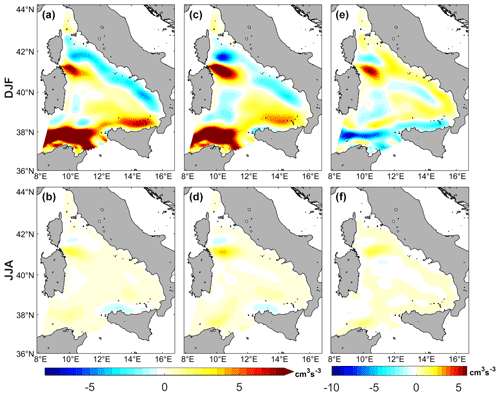
Figure 11Winter (a, c, e) and summer (b, d, f) averages of the wind work (cm3 s−3) taking into account ocean currents averaged from the surface to a depth of 51 m for present (a, b) and future (c, d) climate as computed from ROM. Differences between the wind work in the future and present climate are presented in panels (e) and (f).
As shown in Fig. 11b, at present, the wind work in summer is mainly positive except for the southeast of the Tyrrhenian, where positive and negative side-by-side values appear due to the development of a characteristic summer dynamical structure and in the Bonifacio Gyre (Fig. 3d–f; e.g., de la Vara et al., 2019; Napolitano et al., 2016). It takes smaller values than in winter due to the wind slackening. In the future, the wind work does not present important changes but increases especially in the Bonifacio Gyre area (Fig. 11d and f). The reasoning behind this is that, again, a decrease in the water transport of MAW across the Sardinia Strait increases the effectiveness of the transference of kinetic energy from the wind to these dynamical structures.
4.2 Implications for the water exchange with other basins
We now study how the changes in the water properties across the Tyrrhenian straits in the future can have a possible impact on adjacent areas. In particular, we focus on the Liguro-Provençal basin, the circulation and hydrology of which is strongly affected by waters that exit the Tyrrhenian Sea via the Corsica Channel, and this can have a concomitant effect on the deep convection over the Gulf of Lions (Astraldi et al., 1999).
From the above analysis we can thus conclude that in both the Sardinia Strait and the Corsica Channel the water column presents, qualitatively, the same structure as today, but waters experience a generalized warming which is also observed in the Gulf of Lions (Parras-Berrocal et al., 2021). Also in agreement with that work, surface waters tend to be fresher than today, whilst intermediate-depth waters will be saltier, due a freshening of the Atlantic surface waters that enter the Mediterranean, as well as by an enhanced evaporation that causes intermediate waters to be saltier than today. The decrease in surface salinity in the subpolar North Atlantic and in the eastern limb of the North Atlantic subtropical gyre is a robust feature of CMIP5 multimodel ensemble (Levang and Schmitt, 2020; Soto-Navarro et al., 2020), and the establishment of its causes is a matter of intense research. Very recently Sathyanarayan et al. (2021) point out that besides changes in surface freshwater fluxes, changes in salinity in the Atlantic may be related to changes in wind stress and circulation, which in turn are related to changes in surface warming. Finally, they remark that the projected AMOC (Atlantic Meridional Overturning Circulation) weakening may play an important role. The generalized effect of the warning leads to a density decrease all over the sections studied (Figs. 4 and 5). This reduction is more pronounced at the surface due to the simultaneous warming and freshening, and at intermediate depths, the density reduction is more modest because both temperature and salinity show an increase. A clear implication of this is that the water column becomes more stratified in the future. The waters flowing from the Tyrrhenian towards the Liguro-Provençal basin play a first-order importance role in shaping the stratification of the area and thus in the preconditioning for deep water convection in the Gulf of Lions (e.g., Schroeder et al., 2010). Our results suggest that the combination of the decrease in the volume and the strengthening of the stratification of the waters that flow through the Corsica Channel towards the Liguro-Provençal basin could be one of the contributing factors to the projected interruption of the deep water formation in this area in the future (Margirier et al., 2020; Soto-Navarro et al., 2020).
In this work we examine the possible changes in the Tyrrhenian future surface circulation under the RCP8.5 emission scenario with the atmosphere–ocean regionally coupled model ROM. Special attention is given to the mechanisms that drive the changes by the end of the 21st century and the possible implications in the Liguro-Provençal basin. The Tyrrhenian Sea is a challenging region to study the impact of global warming on the oceanic circulation: it presents complex seasonally dependent circulation patterns and rich mesoscale activity which is controlled by the interaction of local climate and the large-scale Mediterranean circulation through the water transport at its main straits. We show that ROM is capable of successfully reproducing the main features of this circulation. The main conclusions of this study regarding circulation changes in the future can be summarized as follows:
-
In winter, the cyclonic stream around the Tyrrhenian becomes weaker compared to that at present due to a reduced water transport across the Sardinia Strait, while the Bonifacio Gyre and the recirculation area near the Sardinia Strait intensify. This means that the winter pattern becomes less developed than at present, which is consistent with a warming climate.
-
In summer, the dynamical structures become more intense than at present and, as today, the cyclonic stream around the Tyrrhenian Basin breaks down. Therefore, the summer pattern becomes more intensified than at present.
-
The intensification of the dynamical structures in winter is related to the weakening of the cyclonic stream around the Tyrrhenian Sea which is, in turn, caused by a reduction in the inflow of MAW into the basin. This weakening is associated with the increase in the magnitude of the wind work over the main dynamical structures, which are intensified and enlarged.
-
In the future, the northward water transport across the Corsica Channel towards the Liguro-Provençal basin becomes smaller than today. Also, water that flows through this channel presents a stronger stratification than at present. The reason for this is twofold: on the one hand, a freshening of the Atlantic waters inflowing through Gibraltar that causes a reduction of the Mediterranean sea-surface salinity (e.g., Parras-Berrocal et al., 2020) and, on the other hand, a warming with a saltening of intermediate waters. These changes potentially contribute to the interruption of deep water formation in the Gulf of Lions by the end of the century (Parras-Berrocal et al., 2021).
The ROM data are available at https://swiftbrowser.dkrz.de/public/dkrz_64ea1a99-f1de-45dab8d1-a3175f15ee46/ROM_MED_dataset/ (Sein et al., 2015).
The supplement related to this article is available online at: https://doi.org/10.5194/esd-13-303-2022-supplement.
AdlV and WC conceptualized the paper. AdlV conducted the analysis and wrote the manuscript. IMPB prepared the figures and contributed to the manuscript writing. WC participated in the analysis, figure preparation and manuscript writing. AI collaborated with the analysis and manuscript writing. DVS performed the simulations, provided us the data and contributed to the manuscript writing.
The contact author has declared that neither they nor their co-authors have any competing interests.
Publisher's note: Copernicus Publications remains neutral with regard to jurisdictional claims in published maps and institutional affiliations.
We are thankful to the two anonymous referees for their constructive comments on the paper. We acknowledge the use of MATLAB (de la Vara et al., 2022) and CDO (Schulzweida, 2020).
Alba de la Vara and William Cabos have been funded by the Spanish Ministry of Science, Innovation and Universities, the Spanish State Research Agency and the European Regional Development Fund, through grant CGL2017-89583-R. William Cabos has also been supported by Salvador Madariaga grant (Spanish Ministry of Science Innovation and Universities). Iván M. Parras Berrocal was supported through the Spanish National Research Plan through project TRUCO (RTI2018-100865-B-C22). Dmitry V. Sein worked in the framework of the state assignment of the Ministry of Science and Higher Education of Russia (grant no. 0128-2021-0014).
This paper was edited by Yun Liu and reviewed by two anonymous referees.
Artale, V., Astraldi, M., Buffoni, G., and Gasparini, G. P.: Seasonal variability of gyre-scale circulation in the northern Tyrrhenian Sea, J. Geophys. Res., 99, 14127–14137, https://doi.org/10.1016/j.dsr2.2003.08.004, 1994.
Astraldi, M. and Gasparini, G. P.: The seasonal characteristics of the circulation in the North Mediterranean basin and their relationship with the atmospheric-climatic conditions, J. Geophys. Res.-Oceans, 97, 9531–9540, https://doi.org/10.1029/92JC00114, 1992.
Astraldi, M., Balopoulos, S., Candela, J., Font, J., Gacic, M., Gasparini, G. P., Manca, B., Theocharis, A., and Tintoré, J.: The role of straits and channels in understanding the characteristics of Mediterranean circulation, Prog. Oceanogr., 44, 65–108, https://doi.org/10.1016/S0079-6611(99)00021-X, 1999.
Astraldi, M., Gasparini, G. P., Vetrano, A., and Vignudelli, S.: Hydrographic characteristics and interannual variability of water masses in the central Mediterranean: a sensitivity test for long-term changes in the Mediterranean Sea, Deep-Sea Res. Pt. I, 49, 661–680, https://doi.org/10.1016/S0967-0637(01)00059-0, 2002.
Béranger, K., Mortier, L., Gasparini, G. P., Gervasio, L., Astraldi, M., and Crépon, M.: The dynamics of the Sicily Strait: a comprehensive study from observations and models, Deep-Sea Res. Pt. II, 51, 411–440, https://doi.org/10.1016/j.pocean.2004.07.013, 2004.
Béranger, K., Mortier, L., and Crépon, M.: Seasonal variability of water transport through the Straits of Gibraltar, Sicily and Corsica, derived from a high-resolution model of the Mediterranean circulation, Prog. Oceanogr., 66, 341–364, https://doi.org/10.1016/j.pocean.2004.07.013, 2005.
Bergamasco, A. and Malanotte-Rizzoli, P.: The circulation of the Mediterranean Sea: a historical review of experimental investigations, Adv. Oceanogr. Limnol., 1, 11–28, https://doi.org/10.1080/19475721.2010.491656, 2010.
Cabos, W., Sein, D. V., Pinto, J. G., Fink, A. H., Koldunov, N. V., Alvarez, F, Izquierdo, A., Keenlyside, N., and Jacob, D.: The South Atlantic Anticyclone as a key player for the representation of the tropical Atlantic climate in coupled climate model, Clim. Dynam., 48, 4051–4069, https://doi.org/10.1007/s00382-016-3319-9, 2017.
Cabos, W., Sein, D. V., Durán-Quesada, A., Liguori, G., Koldunov, N. K., Martínez-López, B., Alvarez, F., Sieck, K., Limareva, N., and Pinto, J. G.: Dynamical downscaling of historical climate over CORDEX Central America domain with a regionally coupled atmosphere–ocean model, Clim. Dynam., 52, 4305–4328, https://doi.org/10.1007/s00382-018-4381-2, 2018.
Cabos, W., de la Vara, A., Álvarez-García, F. J., Sánchez, E., Sieck, K., Pérez-Sanz, J. I., Limareva, N., and Sein, D. V.: Impact of ocean-atmosphere coupling on regional climate: the Iberian Peninsula case, Clim. Dynam., 54, 4441–4467, https://doi.org/10.1007/s00382-020-05238-x, 2020.
Darmaraki, S., Somot, S., Sevault, F., Nabat, P., Narvaez, W., Cavicchia, L., Djurdjevic, V., Li, L., Sannino, G. M., and Sein, D. V.: Future evolution of marine heat waves in the Mediterranean Sea, Clim. Dynam., 53, 1371–1392, https://doi.org/10.1007/s00382-019-04661-z, 2019.
Dastis, C., Bruno, M., Izquierdo, A., Reyes, E., Sofina, E. V., and Plink, N. L.: Influence of the atmospheric pressure fluctuations over the Mediterranean Sea on the mesoscale water dynamics of the Strait of Gibraltar and the Alboran Sea, Fundamentalnaya i Prikladnaya Gidrofizika, 11, 28–39, https://doi.org/10.7868/S2073667318010033, 2018.
de la Vara, A., Galan del Sastre, P., Arsouze, T., Gallardo, C., and Gaertner, M. A.: Role of atmospheric resolution in the long-term seasonal variability of the Tyrrhenian Sea circulation from a set of ocean hindcast simulations (1997–2008), Ocean Model., 134, 51–67, https://doi.org/10.1016/j.ocemod.2019.01.004, 2019.
de la Vara, A., Parras-Berrocal, I. M., Izquierdo, A., Sein, D. V., and Cabos, W.: Climate change signal in the ocean circulation of the Tyrrhenian Sea, Zenodo [data set], https://doi.org/10.5281/zenodo.5901640, 2022.
Déqué, M., Somot, S., Sanchez-Gomez, E., Goodess, C. M., Jacob, D., Lenderink, G., and Christensen, O. B.: The spread amongst ENSEMBLES regional scenarios: regional climate models, driving general circulation models and interannual variability, Clim. Dynam., 38, 951–964, 2012.
Gasparini, G., Schroeder, K., and Sparnocchia, S.: Straits and Channels as key regions of an integrated marine observatory of the Mediterranean: our experience on their long-term monitoring, Towards an Integrated System of Mediterranean Marine Observatories 34, CIESM Workshop Monographs, 75–79, 2008.
Gérigny, O., Coudray, S., Lapucci, C., Tomasino, C., Bisgambiglia, P. A., and Galgani, F.: Small-scale variability of the current in the Strait of Bonifacio, Ocean Dynam., 65, 1165–1182, https://doi.org/10.1007/s10236-015-0863-5, 2015.
Giorgetta, M. A., Jungclaus, J., Reick, C. H., Legutke, S., Bader, J., Böttinger, M., Brovkin, V.,Crueger, T., Esch, M., Fieg, K., Glushak, K., Gayler, V., Haak, H., Hollweg, H.D., Ilyina, T., Kinne, S., Kornblueh, L., Matei, D., Mauritsen, T., Mikolajewicz, U., Mueller, W., Notz, D., Pithan, F., Raddatz, T., Rast, S., Redler, R., Roeckner, E., Schmidt, H., Schnur, R., Segschneider, J., Six, K. D., Stockhause, M., Timmreck, C., Wegner, J., Widmann, H., Wieners, K. H., Claussen, M., Marotzke, J., and Stevens, B.: Climate and carbon cycle changes from 1850 to 2100 in MPI-ESM simulations for the Coupled Model Intercomparison Project phase 5, J. Adv. Model. Earth Sy., 5, 572–597, https://doi.org/10.1002/jame.20038, 2013.
Giorgi, F.: Climate Change Hot-Spots, Geophys. Res. Lett., 33, L08707, https://doi.org/10.1029/2006GL025734, 2006.
Hagemann, S. and Dümenil Gates, L.: Validation of the hydrological cycle of ECMWF and NCEP reanalyses using the MPI hydrological discharge model, J. Geophys. Res., 106, 1503–1510, 2001.
Hayes, D. R., Schroeder, K., Poulain, P. M., Testor, P., Mortier, L., Bosse, A., and du Madron, X.: 18 Review of the Circulation and Characteristics of Intermediate Water Masses of the Mediterranean: Implications for Cold-Water Coral Habitats, in: Mediterranean Cold-Water Corals: Past, Present and Future, Coral Reefs of the World, vol. 9, edited by: Orejas, C. and Jiménez, C., Springer, Cham, https://doi.org/10.1007/978-3-319-91608-8_18, 2019.
Iacono, R., Napolitano, E., Marullo, S., Artale, V., and Vetrano, A.: Seasonal variability of the Tyrrhenian Sea surface geostrophic circulation as assessed by altimeter data, J. Phys. Oceanogr., 43, 1710–1732, https://doi.org/10.1175/JPO-D-12-0112.1, 2013.
Izquierdo A. and Mikolajewicz, U.: The role of tides in the spreading of Mediterranean Outflow waters along the southwestern Iberian margin, Ocean Model., 133, 27–43, https://doi.org/10.1016/j.ocemod.2018.08.003, 2019.
Jacob, D.: A note to the simulation of the annual and interannual variability of the water budget over the Baltic Sea drainage basin, Meteorol. Atmos. Phys., 77, 61–73, https://doi.org/10.1007/s007030170017, 2001.
Jacob, D. and Podzun, R.: Sensitivity studies with the regional climate model REMO, Meteorol. Atmos. Phys., 63, 119–129, https://doi.org/10.1007/BF01025368, 1997.
Jiménez-Guerrero, P., Montávez, J. P., Domínguez, M., Romera, R., Fita, L., Fernández, J., Cabos, W., Liguori, G., and Gaertner M. A.: Mean fields and interannual variability in RCM simulations over Spain: the ESCENA project, Clim. Res., 57, 201–220, https://doi.org/10.3354/cr01165, 2013.
Jordà, G., Von Schuckmann, K., Josey, S. A., Caniaux, G., García-Lafuente, J., Sammartino, S., Özsoy, E., Polcher, J., Notarstefano, G., Poulain, P. M., Adloff, A., Salat, J., Naranjo, C., Schroeder, K., Chiggiato, J., Sannino, G., and Macías, D.: The Mediterranean Sea heat and mass budgets: estimates, uncertainties and perspectives, Prog. Oceanogr., 156, 174–208, https://doi.org/10.1016/j.pocean.2017.07.001, 2017.
Jungclaus, J. H., Keenlyside, N., Botzet, M., Haak, H., Luo, J. J., Latif, M., Marotzke, J., Mikolajewicz, U., and Roeckner, E.: Ocean circulation and tropical variability in the coupled model ECHAM5/MPI-OM, J. Climate, 19, 3952–3972, https://doi.org/10.1175/JCLI3827.1, 2006.
Jungclaus, J. H., Fischer, N., Haak, H., Lohmann, K., Marotzke, J., Matei, D., Mikolajewicz, U., Notz, D., and von Storch, J. S.: Characteristics of the ocean simulations in MPIOM, the ocean component of the MPI-Earth system model, J. Adv. Model. Earth Sy., 5, 422–446, https://doi.org/10.1002/jame.20023, 2013.
Korres, G., Pinardi, N., and Lascaratos, A.: The ocean response to low-frequency interannual atmospheric variability in the Mediterranean Sea. Part I: sensitivity experiments and energy analysis, J. Climate, 13, 705–731, https://doi.org/10.1175/1520-0442(2000)013<0705:TORTLF>2.0.CO;2, 2000.
Kotlarski, S., Keuler, K., Christensen, O. B., Colette, A., Déqué, M., Gobiet, A., Goergen, K., Jacob, D., Lüthi, D., van Meijgaard, E., Nikulin, G., Schär, C., Teichmann, C., Vautard, R., Warrach-Sagi, K., and Wulfmeyer, V.: Regional climate modeling on European scales: a joint standard evaluation of the EURO-CORDEX RCM ensemble, Geosci. Model Dev., 7, 1297–1333, https://doi.org/10.5194/gmd-7-1297-2014, 2014.
Krivosheya, V. G. and Ovchinnikov, I. M.: Peculiarities in geostrophic circulation of waters of the Tyrrhenian Sea, Oceanology-USSR, 13, 822–827, 1973.
Lascaratos, A., Williams, R. G., and Tragou, E.: A mixed-layer study of the formation of Levantine Intermediate Water, J. Geophys. Res., 98, 14739–14749, https://doi.org/10.1016/0967-0645(93)90064-T, 1993.
Levang, S. J. and Schmitt, R. W.: What Causes the AMOC to Weaken in CMIP5?, J. Climate, 33, 1535–1545, https://doi.org/10.1175/JCLI-D-19-0547.1, 2020.
Limareva, N. S., Cabos, W., Izquierdo, A., and Sein, D. V.: The climate change of the Caucasus as a result of the global warming, Sovremennaa nauka i innovacii, 2, 15–26, 2017.
Liu, F., Mikolajewicz, U., and Six, K.: Drivers of the decadal variability of the North Ionian Gyre upper layer circulation during 1910–2010: a regional modelling study, Clim. Dynam., https://doi.org/10.1007/s00382-021-05714-y, 2021.
Maier-Reimer, E.: Design of a closed boundary regional model of the Arctic Ocean, B. Am. Meteorol. Soc.: Workshop on polar processes in global climate, Cancun, Mexico, 13–15 November 1996, 72–73, 1997.
Margirier, F., Testor, P., Heslop, E., Mallil, K., Bosse, A., Houpert, L., Mortier, L., Bouin, M. N., Coppola, L., D’Ortenzio, F., Durrieu de Madron, X., Mourre, B., Prieur, L., Raimbault, P., and Taillandier, V.: Abrupt warming and salinification of intermediate waters interplays with decline of deep convection in the Northwestern Mediterranean Sea, Sci. Rep., 10, 1–11, https://doi.org/10.1038/s41598-020-77859-5, 2020.
Marsland, S. J., Haak, H., Jungclaus, J. H., Latif, M., and Röske, F.: The Max-Planck-Institute global ocean/sea ice model with orthogonal curvilinear coordinates, Ocean Model., 5, 91–127, https://doi.org/10.1016/S1463-5003(02)00015-X, 2003.
Mathis, M., Elizalde, A., and Mikolajewicz, U.: Which complexity of regional climate system models is essential for downscaling anthropogenic climate change in the Northwest European shelf, Clim. Dynam., 50, 2637–2659, https://doi.org/10.1007/s00382-017-3761-3, 2018.
MEDOC Group: Observation of formation of deep water in the Mediterranean Sea, Nature, 227, 1037–1040, 1970.
Millot, C.: Some features of the Algerian Current, J. Geophys. Res.-Oceans, 90, 7169–7176, https://doi.org/10.1029/JC090iC04p07169, 1985.
Millot, C.: Another description of the Mediterranean Sea outflow, Progr. Oceanogr., 82, 101–124, https://doi.org/10.1016/j.Pocean.2009.04.016, 2009.
Millot, C.: Comments about computations about the Mediterranean Outflow composition, B. Geofis. Teor. Appl., 60, 517–630, https://doi.org/10.4430/bgta0266, 2019.
Napolitano, E., Iacono, R., Sorgente, R., Fazioli, L., Olita, A., Cucco, A., Oddo, P., and Guarnieri, A.: The regional forecasting systems of the Italian seas, J. Oper. Oceanogr., 9, 66–76, https://doi.org/10.1080/1755876X.2015.1117767, 2016.
Ovchinnikov, I. M.: Circulation in the surface and intermediate layers of the Mediterranean, Oceanology, 6, 48–59, 1966.
Parras-Berrocal, I., Vazquez, R., Cabos, W., Sein, D., Alvarez, O., Bruno, M., and Izquierdo, A.: Will deep water formation collapse in the North Western Mediterranean Sea by the end of the 21st century?, Earth and Space Science Open Archive, https://doi.org/10.1002/essoar.10507698.1, 2021.
Parras-Berrocal, I. M., Vazquez, R., Cabos, W., Sein, D., Mañanes, R., Perez-Sanz, J., and Izquierdo, A.: The climate change signal in the Mediterranean Sea in a regionally coupled atmosphere–ocean model, Ocean Sci., 16, 743–765, https://doi.org/10.5194/os-16-743-2020, 2020.
Renault, L., Molemaker, M. J., McWilliams, J. C., Shchepetkin, A. F., Lemarié, F., Chelton, D., Illig, S., and Hall, A.: Modulation of wind work by oceanic current interaction with the atmosphere, J. Phys. Oceanogr., 46, 1685–1704, 2016.
Rinaldi, E., Buongiorno Nardelli, B., Zambianchi, E., Santoleri, R., and Poulain, P. M.: Lagrangian and Eulerian observations of the surface circulation in the Tyrrhenian Sea, J. Geophys. Res., 115, C04024, https://doi.org/10.1029/2009JC005535, 2010.
Rio, M. H., Poulain, P. M., Pascual, A., Mauri, E., Larnicol, G., and Santoleri, R.: A mean dynamic topography of the Mediterranean Sea computed from altimetric data, in-situ measurements and a general circulation model, J. Marine Syst., 65, 484–508, https://doi.org/10.1016/j.jmarsys.2005.02.006, 2007.
Rummukainen, M.: Added value in regional climate modeling, WIREs Clim. Change, 7, 145–159, https://doi.org/10.1002/wcc.378, 2016.
Sathyanarayanan, A., Köhl, A., and Stammer, D.: Ocean Salinity Changes in the Global Ocean under Global Warming Conditions. Part I: Mechanisms in a Strong Warming Scenario, J. Climate, 34, 8219–8236, https://doi.org/10.1175/JCLI-D-20-0865.1, 2021.
Schroeder, K., Josey, S., Herrmann, M., Grignon, L., Gasparini, G., and Bryden, H.: Abrupt warming and salting of the Western Mediterranean deep water after 2005: atmospheric forcings and lateral advection, J. Geophys. Res.-Oceans, 115, C08029, https://doi.org/10.1029/2009JC005749, 2010.
Schulzweida, U.: CDO User Guide (Version 2.0.0), Zenodo [code], https://doi.org/10.5281/zenodo.5614769, 2020.
Sciascia, R., Magaldi, M. G., and Vetrano, A.: Current reversal and associated variability within the Corsica Channel: The 2004 case study, Deep-Sea Res. Pt. I, 144, 39–51, https://doi.org/10.1016/j.dsr.2018.12.004, 2019.
Sein, D. V., Koldunov, N. K., Pinto, J. G., and Cabos, W.: Sensitivity of simulated regional Arctic climate to the choice of coupled model domain, Tellus A, 66, 23966, https://doi.org/10.3402/tellusa.v66.23966, 2014.
Sein, D. V., Mikolajewicz, U., Gröger, M., Fast, I., Cabos, W., Pinto, J. G., Hagemann, S., Semmler, T., Izquierdo, A., and Jacob, D.: Regionally coupled atmosphere-ocean-sea ice-marine biogeochemistry model ROM: 1, J. Adv. Model. Earth Sy., 7, 268–304, https://doi.org/10.1002/2014MS000357, 2015.
Sein, D. V., Gröger, M., Cabos, W., Alvarez-Garcia, F. J., Hagemann, S., Pinto, J. G., Izquierdo, A., de la Vara, A., Koldunov, N., Dvornikov, A., Limareva, N., Alekseeva, E., Martinez-Lopez, B., and Jacob, D.: Regionally coupled atmosphere-ocean-marine biogeochemistry model ROM: 2. Studying the climate change signal in the North Atlantic and Europe, J. Adv. Model. Earth Sy., 12, e2019MS001646, https://doi.org/10.1029/2019MS001646, 2020.
Ser-Giacomi, E., Jordá-Sánchez, G., Sotto-Navarro, J., Thomsen, S., Mignot, J., Sevault, F., and Rossi, V.: Impact of climate change on surface stirring and transport in the Mediterranean Sea, Geophys. Res. Lett., 47, e2020GL089941, https://doi.org/10.1029/2020GL089941, 2020.
Serravall, R. and Cristofalo, G. C.: On the presence of a coastal current of Levantine intermediate water in the central Tyrrhenian Sea, Oceanol. Acta, 22, 281–290, 1999.
Somot, S., Sevault, F., and Déqué, M.: Transient climate change scenario simulation of the Mediterranean Sea for the 21st century using a high resolution ocean circulation model, Clim. Dynam., 27, 851–879, 2006.
Soto-Navarro, J., Jordá, G., Amores, A., Cabos, W., Somot, S., Sevault, F., Macías, D., Djurdjevic, V., Sannino, G., Li, L., and Sein, D.: Evolution of Mediterranean Sea water properties under climate change scenarios in the Med-CORDEX ensemble, Clim. Dynam., 54, 2135–2165, https://doi.org/10.1007/s00382-019-05105-4, 2020.
Taylor, K., Stouffer, R., and Meehl, G.: An overview of CMIP5 and the experiments design, B. Am. Meteorol. Soc., 93, 485–498, https://doi.org/10.1175/BAMS-D-11-00094.1, 2012.
The LIWEX Group: The Levantine Intermediate Water Experiment (LIWEX) Group: Levantine Basin – a laboratory for multiple water mass formation processes, J. Geophys. Res., 108, 8101, https://doi.org/10.1029/2002JC001643, 2003.
Valcke, S., Caubel, A., Declat, D., and Terray, L.: OASIS3 ocean atmosphere sea ice soil user’s guide, 2, CERFACS, Toulouse, France, available at: https://oasis.cerfacs.fr/wp-content/uploads/sites/114/2021/02/GLOBC_TR_oasis3_UserGuide.pdf (last access: 27 January 2022), 2003.
Vargas-Yáñez, M., Plaza, F., Garcıa-Lafuente, J., Sarhan, T., Vargas, J.M, and Vélez-Belchí, P.: About the seasonal variability of the Alboran Sea circulation, J. Marine Syst., 35, 229–248, https://doi.org/10.1016/S0924-7963(02)00128-8, 2002.
Vetrano, A., Gasparini, G. P., Molcard, R., and Astraldi, M.: Water flux estimates in the central Mediterranean Sea from an inverse box model, J. Geophys. Res., 109, C01019, https://doi.org/10.1029/2003JC001903, 2004.
Vetrano, A., Napolitano, E., Iacono, R., Schroeder, K., and Gasparini, G. P.: Tyrrhenian Sea circulation and water mass fluxes in spring 2004: observations and model results, J. Geophys. Res., 115, C06023, https://doi.org/10.1029/2009JC005680, 2010.
Vigo, M. I., Sempere, M. D., Chao, B. F., and Trottini, M.: Mediterranean Surface Geostrophic Circulation from Satellite Gravity and Altimetry Observations, Meteorology and Climatology of the Mediterranean and Black Seas, 269–285, Birkhäuser, Basel, Switzerland, https://doi.org/10.1007/978-3-030-11958-4_16, 2019.
Wüst, G.: On the vertical circulation of the Mediterranean Sea, J. Geophys. Res., 66, 3261–3271, https://doi.org/10.1029/JZ066i010p03261, 1961.
Zavatarelli, M. and Mellor, G. L.: A numerical study of the Mediterranean Sea circulation, J. Phys. Oceanogr., 25, 1384–1414, https://doi.org/10.1175/1520-0485(1995)025<1384:ANSOTM>2.0.CO;2, 1995.