the Creative Commons Attribution 4.0 License.
the Creative Commons Attribution 4.0 License.
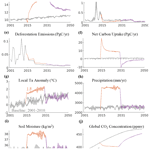
Exploration of a novel geoengineering solution: lighting up tropical forests at night
Xueyuan Gao
Dongdong Wang
Bin He
Aolin Jia
Plants primarily conduct photosynthesis in the daytime, offering an opportunity to increase photosynthesis and carbon sink by providing light at night. We used a fully coupled Earth system model to quantify the carbon sequestration and climate effects of a novel carbon removal proposal: lighting up tropical forests at night via lamp networks above the forest canopy. Simulation results show that additional light increased the tropical forest carbon sink by 10.4±0.05 Pg of carbon per year during a 16-year lighting experiment, resulting in a decrease in atmospheric CO2 and suppression of global warming. In addition, local temperature and precipitation increased. The energy requirement for capturing 1 t of carbon is lower than that of direct air carbon capture. When the lighting experiment was terminated, tropical forests started to release carbon slowly. This study suggests that lighting up tropical forests at night could be an emergency solution to climate change, and carbon removal actions focused on enhancing ecosystem productivity by altering environmental factors in the short term could induce post-action CO2 outgassing.
- Article
(2032 KB) - Full-text XML
-
Supplement
(4967 KB) - BibTeX
- EndNote
Anthropogenic greenhouse gas (GHG) emissions have led the global mean temperature to increase by approximately 1.1 ∘C since the industrial revolution (IPCC, 2013, 2018; IPCC AR6 WGI, 2021). Changes in climate have caused impacts on natural ecosystems and human societies, such as mass ice sheet melt (Jevrejeva et al., 2016), devastating heat waves (Dosio et al., 2018), and an increase in extreme climate events (Kirchmeier-Young and Zhang, 2020), exposing natural and human systems to uncertainties and the risks of unsustainable development (Gao et al., 2019, 2020). Despite the scientific consensus on climate change, emission reduction efforts have made slow or little progress, with global GHG emissions continuing to rise (IPCC AR6 WGI, 2021). In this context, geoengineering options are increasingly being considered as means of deliberately intervening in Earth's climate system in the second half of the 21st century (IPCC AR6 WGI, 2021; Moore et al., 2015).
Existing geoengineering proposals tend to align with two fundamentally different strategies: solar geoengineering (SG) (Abatayo et al., 2020; Proctor et al., 2018; Robock et al., 2009) and carbon capture and sequestration (CCS) (IPCC, 2005; Jones, 2008; Leung et al., 2014). SG and related techniques reduce the amount of incoming radiation from the sun, typically via stratospheric aerosol injection, subsequently affecting the planet's temperature. Although they may be able to offset temperature increase rapidly, previous studies indicate the potential for political instability (Abatayo et al., 2020) and negative impacts on human health (Robock et al., 2009) and agriculture (Proctor et al., 2018). Comparatively, CCS removes carbon from the global carbon cycle by using artificial machines and saves it for long-term storage or for industrial reutilization (IPCC, 2005). While technically feasible, the environmental risks for the transport and storage of CO2, limited carbon storage capability, and high cost remain large obstacles to implementing CCS (IPCC, 2005; Jones, 2008; Leung et al., 2014).
In this study, the authors propose a novel geoengineering solution: lighting up tropical forests at night by installing lamp networks above the forest canopy (Graham et al., 2003), which lengthens photoperiods, leads to greater photosynthesis and carbon sequestration, and helps mitigate climate change. In contrast to traditional CCS techniques, this strategy utilizes a natural carbon sink to capture and sequester CO2 from the air and avoids long-distance transport and geological storage.
Structurally intact tropical forests are by far the most efficient carbon capture method (Mitchard, 2018), and they act as an important carbon sink against rising CO2 levels (Pan et al., 2011; Sullivan et al., 2020). Although intact tropical forest growth is likely suffering from warming and moisture stress induced by anthropogenic greenhouse gas emissions (Aguirre-Gutiérrez et al., 2020; Doughty et al., 2015; Gatti et al., 2021; Hubau et al., 2020), light is still the primary factor limiting tropical tree growth due to cloud cover, especially during the rainy season (Boisvenue and Running, 2006; Graham et al., 2003). Studies on the photoperiodic control of tropical tree growth typically fall into two categories: physiological field observations under seasonal variations of day length (Borchert et al., 2005; Pires et al., 2018; Rivera et al., 2002) and physiological greenhouse observations under experimental variations of the photoperiod (Dixit and Singh, 2014; Djerrab et al., 2021; Luo et al., 2021; Stubblebine et al., 1978). Field observations have shown that longer photoperiods facilitate bud break and flowering in tropical forests (Borchert et al., 2005; Pires et al., 2018; Rivera et al., 2002). Greenhouse experiments either lengthen or shorten photoperiods, and results suggest that short photoperiods reduce plant growth rate and lead to thinner leaves and lower chlorophyll content (Djerrab et al., 2021; Luo et al., 2021), while long photoperiods increase stem growth rate and stimulate tree growth (Dixit and Singh, 2014; Stubblebine et al., 1978). These studies are more focused on specific tropical plant species and tend to agree that longer photoperiods might have a positive effect on vegetative growth in tropical forests. Ecosystem-level field experiments are critical for taking into account key environmental factors that are missing in greenhouse experiments (e.g., water and nutrition constraints) and for informing model parameterizations, although they are lacking so far.
Earth system models provide state-of-the-art computer simulations of key processes and climate states across the Earth (Danabasoglu et al., 2020). In this study the authors used a fully coupled Earth system model, the Community Earth System Model version 2 (CESM2) developed by the US National Center for Atmospheric Research (Danabasoglu et al., 2020), to test the carbon sequestration and climate effects of this geoengineering measure by conducting numerical lighting experiments. Briefly, we added additional diffuse visible light to a tropical forest canopy at night (see Fig. S1 in the Supplement), assuming that trees will receive light from multiple directions (e.g., multiple lamps). Tropical forest grids were defined by “broadleaf evergreen tree area percentage” being greater than 60 % between 20∘ N and 20∘ S. The lighting experiment started from 12:00 UTC on 1 January 2015, and the simulation exercise was conducted across numerous timescales and lighting levels:
-
historical control simulation from 2001 to 2014;
-
24 h lighting experiment with various lighting power on 1 January 2015;
-
16-year lighting experiment with the optimal lighting power from 2015 to 2030;
-
20-year simulation after the experiment termination from 2031 to 2050;
-
future control simulation from 2015 to 2050.
Both experiment and control simulations in the future from 2015 to 2050 were on top of the Shared Socioeconomic Pathway (SSP) 126 scenario (Riahi et al., 2017). Each simulation has a spatial resolution of 1∘ and has two members (created from small perturbations to initial conditions) to provide uncertainty estimation. (See Methods for detailed experimental design.)
The CESM2 is an open-source community coupled model consisting of atmosphere, ocean, land, sea ice, land ice, river, and wave models that exchange states and fluxes via a coupler (Danabasoglu et al., 2020). In this study, we used standard CESM2 configurations and enabled all modules including the Community Atmosphere Model version 6 (CAM6), the Parallel Ocean Program version 2 (POP2) with an ocean biogeochemistry component, the Community Land Model version 5 (CLM5) with a land biogeochemistry component, CICE version 5.1.2 (CICE5), the Community Ice Sheet Model version 2.1 (CISM2.1), the Model for Scale Adaptive River Transport (MOSART), and the NOAA WAVEWATCH III ocean surface wave prediction model version 3.14 (WW3). The CESM2 is part of the Couple Model Intercomparison Project Phase 6 (CMIP6) core simulations and about 20 model intercomparison projects (MIPs) within CMIP6. Extensive evaluation suggests that the CESM2 simulations exhibit agreement with satellite-era observations of the climate mean state, seasonal cycle, and interannual variability, which has identified CESM2 as among the most realistic climate models in the world (Danabasoglu et al., 2020).
2.1 Historical control simulations from 2001 to 2014
CESM2 has published its official historical simulation datasets from 1850–2014 via the Earth System Grid Federation (ESGF; https://esgf-node.llnl.gov/search/cmip6, last access: 27 January 2022). This study analyzed the historical simulation datasets of two members from 2001 to 2014 produced by the CESM2 esm-hist-BPRP case.
2.2 Future experiment and control simulations from 2015 to 2050
The selection of 2015 as the start year of the lighting experiment follows CMIP6 future scenario simulation rules. The future experiment simulations and control simulations were both based on the Shared Socioeconomic Pathway (SSP) 126 scenario (Riahi et al., 2017), which is a low-emission (low fossil fuel combustion and deforestation) scenario. The Earth's climate state under SSP126 is close to the current climate state with respect to high-emission scenarios. Therefore, the selection of SSP126 controlled variables and allowed us to see how the lighting experiment influences tropical forest carbon fluxes and climate. This study ran the CESM2 esm-SSP126-BPRP case with the official restart files from historical simulations (esm-hist-BPRP case). Thus, no model spin-up was needed. All simulations were forced with specified greenhouse gas emissions rather than atmospheric greenhouse gas concentrations, so the atmospheric CO2 concentration was prognostic and land and ocean carbon cycles feed back on atmospheric CO2. Each simulation has a nominal horizontal resolution of 1∘ and has two members created from small perturbations to initial climate states to estimate uncertainties.
2.3 The lighting experiment design
The authors modified the radiation module (Rapid Radiative Transfer Model for General circulation models, RRTMG) of CESM2 to add diffuse visible light to a tropical forest canopy at night. CESM2 determines if a grid column is at daytime or nighttime by calculating its cosine (solar zenith angle) at each time step. A negative cosine indicates the grid column is at nighttime and the incoming solar radiation would be assigned with zero. A positive cosine indicates daytime, and the cosine value would be used to calculate incoming solar radiation. The land module then calculates and passes the surface albedo to the atmosphere module, and the atmosphere module calculates the radiation fluxes with the surface albedo and the model-calculated incoming solar radiation. We made modifications in all active modules to switch the sign of tropical forests' cosine from negative to positive when tropical forests were at night. As a result, all modules regarded tropical forests to be at daytime at every time step.
CESM2 divides the incoming solar radiation into four components: direct visible light, diffuse visible light, direct near-infrared (NIR) light, and diffuse near-infrared light. The authors assume that the artificial light would be provided by a lamp network above the forest canopy and that trees receive light from multiple directions. Therefore, the artificial light was specified as diffuse visible light for simplification. In the model, we assigned the diffuse visible light component of the incoming solar radiation with 100, 200, 300, or 400 and other components with 0. The surface albedo was still calculated by the land module and passed to the atmosphere module. The radiation fluxes were then calculated by the model-calculated surface albedo and manually specified solar insolation.
2.4 The calculation of the energy requirement for capturing 1 t of carbon
Here, E is the energy requirement for capturing 1 t of carbon per year, power is 200 W m−2 (nighttime lighting power), area is the tropical forest area of 10.71×1010 m2 (CESM2 output), “hours” represents the amount of nighttime lighting hours per year (365×11), and carbon is the net carbon uptake per year (Fig. 2f) simulated by CESM2. There are no assumed data in this calculation.
3.1 24 h lighting experiment with various lighting power on 1 January 2015
Figure 1 shows the changes in carbon and energy fluxes of Amazonian tropical forests for 24 h since the start of the nighttime lighting experiment at 12:00 UTC on 1 January 2015 (see Figs. S2 and S3 for African and Asian tropical forest responses). Tropical forests had a significant response to nighttime radiation, but the response was different under 100, 200, 300, and 400 W m−2 lighting power. The lighting experiment altered the nighttime energy balance and increased near-surface air temperature, latent heat, and sensible heat. Higher lighting power led to greater increases in air temperature, latent heat, and sensible heat. Meanwhile, the additional light activated photosynthesis and increased net ecosystem productivity (NEP). Nighttime NEP reached the peak at 200 W m−2 and seemed to be suppressed when the lighting power was higher. Comparison of NEP across lighting power suggests that 200 W m−2 is optimal in terms of activating additional photosynthesis. The nighttime NEP is higher than daytime because nighttime surface radiation is solely diffuse visible light, while daytime surface radiation is composed of direct NIR (∼16 %), diffuse NIR (∼30 %), direct visible light (∼15 %), and diffuse visible light (∼39 %). African and Asian tropical forests showed similar responses.
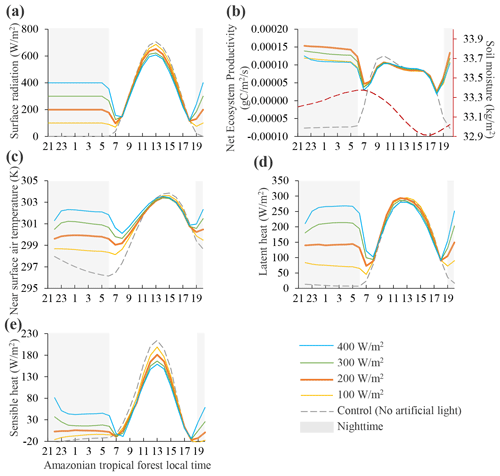
Figure 1Amazonian tropical forest responses for 24 h since the start of the nighttime lighting experiment at 12:00 UTC on 1 January 2015 under various nighttime lighting power. Panel (a) refers to surface downward shortwave radiation. Nighttime NEP (b) reached the peak at 200 W m−2, suggesting that 200 W m−2 is optimal in terms of activating additional photosynthesis.
During daytime in the control simulation, the maximum NEP is at around 09:00–11:00 LT (Fig. 1b). It is not likely to be due to clouds according to the diurnal pattern of the surface downward shortwave radiation (Fig. 1a). We examined the diurnal curve of the soil moisture (the red dashed line in Fig. 1b), and it seems to be due to soil moisture stress. Soil moisture was consumed quickly in the morning, which led to water stress for plant growth in the afternoon. The soil moisture pattern also explains the biased distribution of daytime surface air temperature (Fig. 1c), as well as slightly biased daytime latent heat (Fig. 1d) and daytime sensible heat (Fig. 1e).
3.2 16-year lighting experiment with optimal lighting power from 2015 to 2030
The yellow lines in Fig. 2 show that tropical forest carbon fluxes and climates were significantly altered by a 16-year continuous lighting experiment at night with 200 W m−2 power. The annual gross primary production and autotrophic respiration doubled nearly instantaneously, while the heterotrophic respiration had a slower response and increased continuously over a longer period. We purport these changes to be due to the increase in local temperature and the gradual accumulation of organic matter in the soil. Simulation results show that the lighting experiment also decreased wildfire emissions as soil moisture increased despite the expansion of the coarse woody debris and litter carbon pool that provides potential burning materials. Overall, the net carbon uptake increased to around 25 Pg of carbon per year (Pg C yr−1) at the beginning of the lighting experiment, although it decreased with time due to the continuous increase in heterotrophic respiration. The lighting experiment increased the net carbon uptake in tropical forests by 15.3 times over the simulation period (from 0.68±0.02 Pg C yr−1 over 2001–2014 to 11.1±0.05 Pg C yr−1 over 2015–2030). Among all the absorbed carbon, 75 % entered the vegetation carbon pool, 16 % entered the coarse woody debris and litter carbon pool, and 9 % entered the soil carbon pool (Fig. 3b).
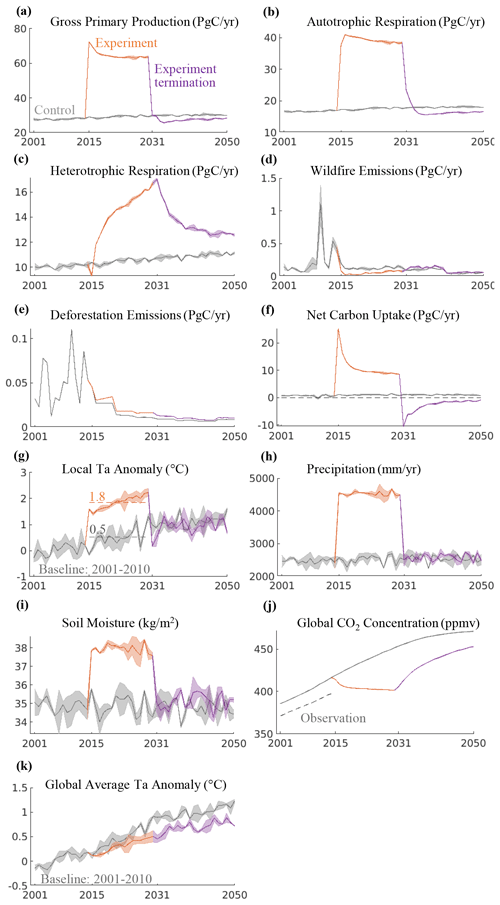
Figure 2Global tropical forest carbon flux and climate responses during and after the lighting experiment. “Ta” in panels (g, k) represents near-surface air temperature. Soil moisture in panel (i) refers to the mass of water in the 10 cm soil surface. Shaded areas represent uncertainties.
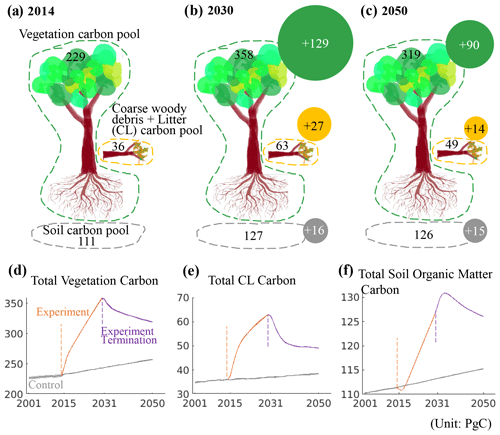
Figure 3Where did the net absorbed carbon go? Global tropical forest carbon amount responses. (a) The current carbon amount in different carbon pools. (b) Carbon amount in 2030 after 16-year lighting experiments. (c) Carbon amount in 2050 after 20 years since the termination of the lighting experiments. The solid circles in panels (b) and (c) refer to carbon amount changes with respect to panel (a). The numbers in panels (a)–(c) are based on panels (d)–(f). Tree drawing courtesy of © Ning Zeng.
Simulation results show that local climates were also significantly impacted (Fig. 2g and h). The annual average air temperature increased by around 1.3∘, and annual precipitation almost doubled. The temperature and precipitation increase showed no significant seasonal trend (Fig. S4). Globally, the atmospheric CO2 concentration dropped quickly in the first several years, while it turned flat in the latter part of the lighting experiment. As a result, the global average air temperature increase was suppressed by around 0.5∘.
Amazonian, African, and Asian tropical forests present different capabilities to offset annual atmospheric carbon accumulation during the lighting experiment (Fig. 4). In the current global carbon budget (Friedlingstein et al., 2019) (averaged from 2009 to 2018), approximately 11±0.5 Pg C yr−1 was released into the atmosphere by anthropogenic activities including fossil fuel combustion and land use, among which 2.5±0.6 Pg C yr−1 was absorbed by the ocean, 3.2±0.6 Pg C yr−1 was absorbed by land, and 4.9±0.02 Pg C yr−1 was accumulated in the atmosphere, resulting in concerns about warming and climate change. The lighting experiment enhanced Amazonian tropical forest net carbon uptake to 6.5±0.04 Pg C yr−1 (averaged during 2015 to 2030), suggesting that lighting up Amazonian tropical forests along could completely offset anthropogenic carbon emissions. African and Asian tropical forests showed lower capabilities, with the net carbon uptake being approximately 2.0±0.002 and 2.6±0.008 Pg C yr−1, respectively (see Figs. S5–S7 for Amazonian, African, and Asian tropical forest carbon flux, carbon amount, and climate responses, respectively).
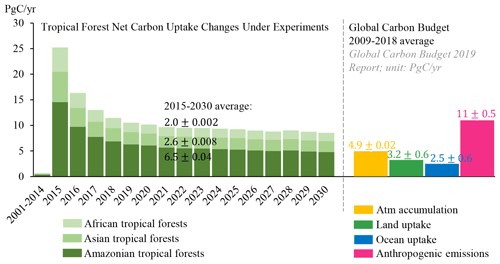
Figure 4Capabilities of Amazonian, African, and Asian tropical forests to offset annual atmospheric carbon accumulation.
We estimated the energy requirement of this strategy for capturing 1 t of carbon (see Methods) and compared it to that of direct air carbon capture (DACC) estimated by recent studies (Chatterjee and Huang, 2020; Realmonte et al., 2019). As the carbon uptake efficiency of the tropical forest ecosystem decreases with time when under consecutive nighttime lighting, the energy requirement for capturing 1 t of carbon increases (Fig. 5, purple line). Nevertheless, the energy requirement of this strategy is lower than that of DACC or is equivalent to the most optimistic estimation of DACC's energy requirement that excludes the energy costs required for carbon transport, storage, and utilization (see Discussion).
3.3 20-year simulation after the experiment termination from 2031 to 2050
The lighting experiment was terminated at 12:00 UTC on 1 January 2031, and model simulations continued for 20 years to 2050 (see the purple lines in Fig. 2). The annual gross primary production and autotrophic respiration dropped quickly, ultimately reaching levels that were even lower than the control period due to a reduction in atmospheric CO2 (CO2 has a fertilization effect in the model). Heterotrophic respiration remained high and decreased much slower at a speed 10 times lower than gross primary production and autotrophic respiration. The soil organic matter carbon pool continued to expand due to the entering of litter carbon during the first 2–3 years following the experiment termination (Fig. 3f). The vegetation carbon pool shrunk as trees produced fewer leaves (Fig. 3d). As a result, tropical forests turned into a net carbon source and remained so until the end of the simulation in 2050 (Fig. 2f). A total of 31.4 % of the carbon that had been absorbed during the lighting experiment was released back to the atmosphere. This number would likely be higher if the simulation continued. As a result, the global atmospheric CO2 concentration returned to a level slightly lower than the control scenario. Local air temperature and precipitation returned to control levels.
Physiological responses of tropical trees to longer photoperiods at the ecosystem level remain among the biggest uncertainties in model simulations. Some field experiments indicate that higher CO2 did not increase carbon sequestration of forests without added nutrients (Oren et al., 2001), suggesting tree growth might be limited by nutrient supply. The simulated local warming might also suppress tree growth (Gatti et al., 2021). Some observational evidence shows that intact tropical forest carbon sinks have been negatively influenced by warming and moisture stress (Doughty et al., 2015; Gatti et al., 2021) and might be reaching saturation (Hubau et al., 2020). However, the model-predicted increases in precipitation and soil moisture, as well as previous studies, have shown that hydroclimate plays a key role in deciding the effects of warming on tree growth (Guan et al., 2015; Reich et al., 2018). No direct evidence exists to verify the simulation results. Ecosystem-level field experiments are needed to understand how tropical forest ecosystems respond to longer photoperiods.
CESM2 likely overestimated the local air temperature increase in tropical forests for the omission of chemical energy stored during photosynthesis (Sellers, 1992). In CESM2 and other modern Earth system models (Sellers, 1992), the canopy energy equation (Danabasoglu et al., 2020) uses the solar radiation absorbed by the vegetation to calculate temperature:
where Sv is the solar radiation absorbed by the vegetation, Lv is the net longwave radiation absorbed by vegetation, and Hv and λEv are the sensible and latent heat fluxes from vegetation, respectively. Lv, Hv, and λEv depend on the vegetation temperature Tv.
The chemical energy that is stored during photosynthesis and released by respiration is ignored as the net chemical energy usually amounts to less than 1 % of absorbed insolation (around 0.6 %; Trenberth et al., 2009). In our lighting experiment from 2015 to 2030, however, 17 % of absorbed insolation was fixed in the ecosystem as chemical energy (Fig. 2f) and did not contribute to local air temperature increase. The model failed to exclude this chemical energy storage from the energy equation. Therefore, the model overestimated the local temperature increase. This suggests that the temperature simulation results should be treated carefully when Earth system models are used to do extreme scenario experiments associated with biogeochemistry.
Tropical forests experienced a significant increase in carbon sink during the lighting experiment but ultimately transitioned from a sink to a source after the experiment was terminated (Fig. 2f). Studies (Koven et al., 2021; Tokarska and Zickfeld, 2015) investigating the effects of overshoot future scenarios (positive carbon emissions followed by net negative emissions) on the terrestrial carbon cycle have observed a similar phenomenon. During a positive emissions phase, terrestrial carbon cycles tend to absorb some fraction of added CO2; however, during a removal phase they tend to release CO2. The mechanism of these phenomena is the different responding rates of vegetative primary productivity and heterotrophic respiration to lengthening and shortening photoperiods or increasing and decreasing atmospheric CO2, with primary productivity responding much quicker than heterotrophic respiration. It is understandable when considering the diurnal pattern of forest carbon uptake. In the daytime, forests act as a carbon sink because photosynthesis is greater than respiration. In the nighttime respiration continues while photosynthesis abates, making forests a carbon source. Additional light and CO2 would increase carbon sink by increasing both photosynthesis and respiration (sometimes referred to as a fertilization effect). When the additional light and CO2 are removed, photosynthesis decreases quickly while respiration remains high, making forests a greater carbon source. It suggests that carbon removal actions focused on enhancing ecosystem productivity by altering environmental factors in the short term could induce this post-action CO2 outgassing.
The global average surface air temperature remained below the control level after the termination of lighting experiments due to a reduction in atmospheric CO2 concentration (Fig. 2k). However, the local air temperature went back to the control level and does not seem to be influenced by CO2 reduction (Fig. 2g). We attribute this to two possible factors. First, different regions tend to have diverse air temperature responses to global CO2 changes. Arctic regions show a much larger temperature increase in response to CO2 increase, while the temperature increase in tropical regions is not that significant. Similarly, the CO2 reduction may have diverse impacts on temperature changes in different regions. Second, the temperature change in tropical forests at the termination of the experiment is controlled by two factors in this study: decreased incoming shortwave radiation and reduced CO2. The former has a much larger impact on the local energy balance than the latter. Therefore, the influence of CO2 reduction on local tropical forests is not as large as on the global scale.
Large clean energy requirements have always been a hurdle to large-scale deployment of any carbon dioxide removal (CDR) techniques, including DACC and the strategy we discuss in this study. Our estimation suggests that the energy requirement of this strategy for capturing 1 t of carbon is less than that of DACC. Specifically, if we give DACC 100 units of energy (100 MWh) per year, DACC could fix 3–12 t carbon per year. If we give forests 100 units of extra energy per year, forests could fix around 19.5 t carbon per year on average (15-year average: 29 t carbon in the first year and 10 t carbon in the 15th year due to an increase in soil respiration); however, only 17 units of energy are actively used to fix carbon, and the remaining 83 units of energy end up as heat, which increases local temperature. Therefore, the energy use efficiency of this strategy is low, which is a major drawback.
Other than the direct lighting energy, this strategy requires additional energy associated with manufacturing and installing lamp networks, constructing electricity transmission devices, and so forth. To make a direct comparison to DACC, we only focus on the energy requirement specifically for carbon capture. Therefore, we did not include the energy costs associated with engineering aspects, as the estimation of DACC's energy requirement does not include the energy costs required for carbon transport, storage, and utilization. In this study, we also mainly focus on the physical understanding of tropical forest ecosystem responses to nighttime artificial lighting, so we did not have much discussion on engineering aspects (how such a network of lamps could be constructed) or cost estimates. Nevertheless, the estimation of additional energy costs and the engineering feasibility are important, and we will attempt to address these issues in future studies.
As to the energy source, we assume this strategy only uses clean energy coming from solar, wind, or nuclear farms to avoid extra carbon emissions when providing light to forests. In terms of technical analysis, more clean energy can be acquired by deploying more low-carbon energy-generation plants across the globe (e.g., building large-scale solar and wind farms in the Sahara; Li et al., 2018). In terms of economic analysis, however, both DACC and this strategy are energetically and financially costly and are therefore unrealistic at present (Chatterjee and Huang, 2020). Moreover, even if the clean-energy-generation capacity increases, we cannot expect the global clean energy supply to only be invested in absorbing CO2. Nevertheless, if society has urgency to intervene in Earth's climate by removing CO2 from the atmosphere in the late half of the 21th century and/or an energy revolution is established and we achieve a significant surplus of clean energy, CDR would still be a powerful and effective climate mitigation strategy.
Another critical negative impact of this strategy is the potential threat to local wildlife and biodiversity. Tropical forests are the repository of a large proportion of Earth's biodiversity, and many of the organisms in the tropics are nocturnal or crepuscular, with organisms and interactions occurring in darkness. An extension of photoperiods could disrupt the habits of nocturnal creatures and have unexpectedly large impacts on ecosystem biodiversity. In addition, the disruption, disturbance, and habitat fragmentation resulting from installing lights throughout tropical forests and throughout the forest canopies could exacerbate the negative impacts of this strategy. Given the potentially inverse relationship between more light at night and ecosystem health, policy makers may consider extending the photoperiod to an appropriate level to increase carbon sequestration while protecting local biodiversity from disastrous impacts. The trade-off between nighttime carbon sequestration and biodiversity preservation should be rigorously evaluated and weighed in the decision-making process.
Overall, lighting up tropical forests at night has led to a significant increase in carbon uptake, a decrease in atmospheric CO2 concentration, and suppression of global warming as simulated by an Earth system model. However, it has strong side effects after the termination of nighttime lighting. In addition, local ecosystem changes could have negative impacts on local wildlife. Practical issues include the large demand for clean energy and the difficulties of implementation. From a positive viewpoint it might be treated as an emergency climate solution if society relies heavily on carbon removal to adjust the Earth's climate in the future. The Paris Agreement set climate goals to limit global warming to well below 2 ∘C and preferably to 1.5 ∘C compared to preindustrial levels (Lawrence et al., 2018). To accomplish the Paris Agreement's climate goals, different engineering levels (lighting power, areas, and periods) might be needed under various anthropogenic emission scenarios, with high-emission scenarios possibly requiring high engineering levels. This study investigated the highest engineering level (lighting up global tropical forests at night with the optimal power) under a low-emission scenario (see Methods). Further research is needed to investigate the relationship between engineering levels and emission scenarios in the context of global climate goals set out by the Paris Agreement (Lawrence et al., 2018).
Current geoengineering studies mainly focus on the evaluation of climate goals that a potential solution might or might not accomplish; however, the changes in Earth's climate after terminating a geoengineering measure tend to be overlooked. This study suggests the importance of post-geoengineering analysis in geoengineering studies.
CESM2 is an open-source community climate model preserved at https://doi.org/10.1029/2019MS001916 (Danabasoglu et al., 2020). All data have been included in the paper.
The supplement related to this article is available online at: https://doi.org/10.5194/esd-13-219-2022-supplement.
XG designed the study and performed the simulations. XG, SL, DW, YL, BH, and AJ contributed to the data interpretation. XG drafted the original version of the paper. SL and DW reviewed and edited the paper.
The contact author has declared that neither they nor their co-authors have any competing interests.
Publisher's note: Copernicus Publications remains neutral with regard to jurisdictional claims in published maps and institutional affiliations.
We would like to acknowledge high-performance computing support from Cheyenne (https://doi.org/10.5065/D6RX99HX) provided by NCAR's Computational and Information Systems Laboratory, sponsored by the National Science Foundation. We would like to acknowledge the constructive comments and suggestions from William Wieder.
This paper was edited by Ben Kravitz and reviewed by Jessica Gurevitch and one anonymous referee.
Abatayo, A. Lou, Bosetti, V., Casari, M., Ghidoni, R., and Tavoni, M.: Solar geoengineering may lead to excessive cooling and high strategic uncertainty, P. Natl. Acad. Sci. USA, 117, 13393–13398, https://doi.org/10.1073/pnas.1916637117, 2020.
Aguirre-Gutiérrez, J., Malhi, Y., Lewis, S. L., Fauset, S., Adu-Bredu, S., Affum-Baffoe, K., Baker, T. R., Gvozdevaite, A., Hubau, W., Moore, S., Peprah, T., Ziemińska, K., Phillips, O. L., and Oliveras, I.: Long-term droughts may drive drier tropical forests towards increased functional, taxonomic and phylogenetic homogeneity, Nat. Commun., 11, 3–18, https://doi.org/10.1038/s41467-020-16973-4, 2020.
Boisvenue, C. and Running, S. W.: Impacts of climate change on natural forest productivity - Evidence since the middle of the 20th century, Global Change Biol., 12, 862–882, https://doi.org/10.1111/j.1365-2486.2006.01134.x, 2006.
Borchert, R., Renner, S. S., Calle, Z., Havarrete, D., Tye, A., Gautier, L., Spichiger, R., and Von Hildebrand, P.: Photoperiodic induction of synchronous flowering near the Equator, Nature, 433, 627–629, https://doi.org/10.1038/nature03259, 2005.
Chatterjee, S. and Huang, K. W.: Unrealistic energy and materials requirement for direct air capture in deep mitigation pathways, Nat. Commun., 11, 4–9, https://doi.org/10.1038/s41467-020-17203-7, 2020.
Danabasoglu, G., Lamarque, J. F., Bacmeister, J., Bailey, D. A., DuVivier, A. K., Edwards, J., Emmons, L. K., Fasullo, J., Garcia, R., Gettelman, A., Hannay, C., Holland, M. M., Large, W. G., Lauritzen, P. H., Lawrence, D. M., Lenaerts, J. T. M., Lindsay, K., Lipscomb, W. H., Mills, M. J., Neale, R., Oleson, K. W., Otto-Bliesner, B., Phillips, A. S., Sacks, W., Tilmes, S., van Kampenhout, L., Vertenstein, M., Bertini, A., Dennis, J., Deser, C., Fischer, C., Fox-Kemper, B., Kay, J. E., Kinnison, D., Kushner, P. J., Larson, V. E., Long, M. C., Mickelson, S., Moore, J. K., Nienhouse, E., Polvani, L., Rasch, P. J., and Strand, W. G.: The Community Earth System Model Version 2 (CESM2), J. Adv. Model. Earth Syst., 12, 78–94, https://doi.org/10.1029/2019MS001916, 2020.
Dixit, A. S. and Singh, N. S.: Photoperiodic control of testicular growth, histomorphology and serum testosterone levels in the male Eurasian tree sparrow: Involvement of circadian rhythm, Gen. Comp. Endocrinol., 208, 5–11, https://doi.org/10.1016/j.ygcen.2014.09.003, 2014.
Djerrab, D., Bertrand, B., Breitler, J. C., Léran, S., Dechamp, E., Campa, C., Barrachina, C., Conejero, G., Etienne, H., and Sulpice, R.: Photoperiod-dependent transcriptional modifications in key metabolic pathways in Coffea arabica, Tree Physiol., 41, 302–316, https://doi.org/10.1093/treephys/tpaa130, 2021.
Dosio, A., Mentaschi, L., Fischer, E. M., and Wyser, K.: Extreme heat waves under 1.5 ∘C and 2 ∘C global warming, Environ. Res. Lett., 13, 234–246, https://doi.org/10.1088/1748-9326/aab827, 2018.
Doughty, C. E., Metcalfe, D. B., Girardin, C. A. J., Amézquita, F. F., Cabrera, D. G., Huasco, W. H., Silva-Espejo, J. E., Araujo-Murakami, A., Da Costa, M. C., Rocha, W., Feldpausch, T. R., Mendoza, A. L. M., Da Costa, A. C. L., Meir, P., Phillips, O. L., and Malhi, Y.: Drought impact on forest carbon dynamics and fluxes in Amazonia, Nature, 519, 78–82, https://doi.org/10.1038/nature14213, 2015.
Friedlingstein, P., Jones, M. W., O'Sullivan, M., Andrew, R. M., Hauck, J., Peters, G. P., Peters, W., Pongratz, J., Sitch, S., Le Quéré, C., Bakker, D. C. E., Canadell1, J. G., Ciais1, P., Jackson, R. B., Anthoni1, P., Barbero, L., Bastos, A., Bastrikov, V., Becker, M., Bopp, L., Buitenhuis, E., Chandra, N., Chevallier, F., Chini, L. P., Currie, K. I., Feely, R. A., Gehlen, M., Gilfillan, D., Gkritzalis, T., Goll, D. S., Gruber, N., Gutekunst, S., Harris, I., Haverd, V., Houghton, R. A., Hurtt, G., Ilyina, T., Jain, A. K., Joetzjer, E., Kaplan, J. O., Kato, E., Goldewijk, K. K., Korsbakken, J. I., Landschützer, P., Lauvset, S. K., Lefèvre, N., Lenton, A., Lienert, S., Lombardozzi, D., Marland, G., McGuire, P. C., Melton, J. R., Metzl, N., Munro, D. R., Nabel, J. E. M. S., Nakaoka, S. I., Neill, C., Omar, A. M., Ono, T., Peregon, A., Pierrot, D., Poulter, B., Rehder, G., Resplandy, L., Robertson, E., Rödenbeck, C., Séférian, R., Schwinger, J., Smith, N., Tans, P. P., Tian, H., Tilbrook, B., Tubiello, F. N., Van Der Werf, G. R., Wiltshire, A. J., and Zaehle, S.: Global carbon budget 2019, Earth Syst. Sci. Data, 11, 1783–1838, https://doi.org/10.5194/essd-11-1783-2019, 2019.
Gao, X., Liang, S., and He, B.: Detected global agricultural greening from satellite data, Agr. Forest Meteorol., 276–277, 1–8, https://doi.org/10.1016/j.agrformet.2019.107652, 2019.
Gao, X., Liang, S., and Sauer, J.: Greening Hiatus in Eurasian Boreal Forests Since 1997 Caused by a Wetting and Cooling Summer Climate, J. Geophys. Res.-Biogeo., 125, e2020JG005662, https://doi.org/10.1029/2020JG005662, 2020.
Gatti, L. V., Basso, L. S., Miller, J. B., Gloor, M., Gatti Domingues, L., Cassol, H. L. G., Tejada, G., Aragão, L. E. O. C., Nobre, C., Peters, W., Marani, L., Arai, E., Sanches, A. H., Corrêa, S. M., Anderson, L., Von Randow, C., Correia, C. S. C., Crispim, S. P., and Neves, R. A. L.: Amazonia as a carbon source linked to deforestation and climate change, Nature, 595, 388–393, https://doi.org/10.1038/s41586-021-03629-6, 2021.
Graham, E. A., Mulkey, S. S., Kitajima, K., Phillips, N. G., and Wright, S. J.: Cloud cover limits net CO2 uptake and growth of a rainforest tree during tropical rainy seasons, P. Natl. Acad. Sci. USA, 100, 572–576, https://doi.org/10.1073/pnas.0133045100, 2003.
Guan, K., Pan, M., Li, H., Wolf, A., Wu, J., Medvigy, D., Caylor, K. K., Sheffield, J., Wood, E. F., Malhi, Y., Liang, M., Kimball, J. S., Saleska, S. R., Berry, J., Joiner, J., and Lyapustin, A. I.: Photosynthetic seasonality of global tropical forests constrained by hydroclimate, Nat. Geosci., 8, 284–289, https://doi.org/10.1038/ngeo2382, 2015.
Hubau, W., Lewis, S. L., Phillips, O. L., Affum-Baffoe, K., Beeckman, H., Cuní-Sanchez, A., Daniels, A. K., Ewango, C. E. N., Fauset, S., Mukinzi, J. M., Sheil, D., Sonké, B., Sullivan, M. J. P., Sunderland, T. C. H., Taedoumg, H., Thomas, S. C., White, L. J. T., Abernethy, K. A., Adu-Bredu, S., Amani, C. A., Baker, T. R., Banin, L. F., Baya, F., Begne, S. K., Bennett, A. C., Benedet, F., Bitariho, R., Bocko, Y. E., Boeckx, P., Boundja, P., Brienen, R. J. W., Brncic, T., Chezeaux, E., Chuyong, G. B., Clark, C. J., Collins, M., Comiskey, J. A., Coomes, D. A., Dargie, G. C., de Haulleville, T., Kamdem, M. N. D., Doucet, J. L., Esquivel-Muelbert, A., Feldpausch, T. R., Fofanah, A., Foli, E. G., Gilpin, M., Gloor, E., Gonmadje, C., Gourlet-Fleury, S., Hall, J. S., Hamilton, A. C., Harris, D. J., Hart, T. B., Hockemba, M. B. N., Hladik, A., Ifo, S. A., Jeffery, K. J., Jucker, T., Yakusu, E. K., Kearsley, E., Kenfack, D., Koch, A., Leal, M. E., Levesley, A., Lindsell, J. A., Lisingo, J., Lopez-Gonzalez, G., Lovett, J. C., Makana, J. R., Malhi, Y., Marshall, A. R., Martin, J., Martin, E. H., Mbayu, F. M., Medjibe, V. P., Mihindou, V., Mitchard, E. T. A., Moore, S., Munishi, P. K. T., Bengone, N. N., Ojo, L., Ondo, F. E., Peh, K. S. H., Pickavance, G. C., Poulsen, A. D., Poulsen, J. R., Qie, L., Reitsma, J., Rovero, F., Swaine, M. D., Talbot, J., Taplin, J., Taylor, D. M., Thomas, D. W., Toirambe, B., Mukendi, J. T., Tuagben, D., Umunay, P. M., van der Heijden, G., Verbeeck, H., Vleminckx, J., Willcock, S., Wöll, H., Woods, J., and Zemagho, L.: Asynchronous carbon sink saturation in African and Amazonian tropical forests, Nature, 579, 80–87, https://doi.org/10.1038/s41586-020-2035-0, 2020.
IPCC: The IPCC special report on carbon dioxide capture and storage, New York, available at: https://www.ipcc.ch/report/carbon-dioxide-capture-and-storage/ (last access: 27 January 2022), 2005.
IPCC: Climate Change 2013: The Physical Science Basis, available at: https://www.ipcc.ch/report/ar5/wg1/ (last access: 27 January 2022), 2013.
IPCC: Global Warming of 1.5 ∘C, available at: https://www.ipcc.ch/sr15/ (last access: 27 January 2022), 2018.
IPCC AR6 WGI: Climate Change 2021: The Physical Science Basis, available at: https://www.ipcc.ch/report/sixth-assessment-report-working-group-i/ (last access: 27 January 2022), 2021.
Jevrejeva, S., Jackson, L. P., Riva, R. E. M., Grinsted, A., and Moore, J. C.: Coastal sea level rise with warming above 2 ∘C, P. Natl. Acad. Sci. USA, 113, 13342–13347, https://doi.org/10.1073/pnas.1605312113, 2016.
Jones, N.: Sucking carbon out of the air, Nature, 459, 228–231, https://doi.org/10.1038/news.2008.1319, 2008.
Kirchmeier-Young, M. C. and Zhang, X.: Human influence has intensified extreme precipitation in North America, P. Natl. Acad. Sci. USA, 117, 13308–13313, https://doi.org/10.1073/pnas.1921628117, 2020.
Koven, C., Arora, V. K., Cadule, P., Fisher, R. A., Jones, C. D., Lawrence, D. M., Lewis, J., Lindsey, K., Mathesius, S., Meinshausen, M., Mills, M., Nicholls, Z., Sanderson, B. M., Swart, N. C., Wieder, W. R., and Zickfeld, K.: 23rd Century surprises: Long-term dynamics of the climate and carbon cycle under both high and net negative emissions scenarios, Earth Syst. Dynam. Discuss. [preprint], https://doi.org/10.5194/esd-2021-23, in review, 2021.
Lawrence, M. G., Schäfer, S., Muri, H., Scott, V., Oschlies, A., Vaughan, N. E., Boucher, O., Schmidt, H., Haywood, J., and Scheffran, J.: Evaluating climate geoengineering proposals in the context of the Paris Agreement temperature goals, Nat. Commun., 9, 1–7, https://doi.org/10.1038/s41467-018-05938-3, 2018.
Leung, D. Y. C., Caramanna, G., and Maroto-Valer, M. M.: An overview of current status of carbon dioxide capture and storage technologies, Renew. Sustain. Energy Rev., 39, 426–443, https://doi.org/10.1016/j.rser.2014.07.093, 2014.
Li, Y., Kalnay, E., Motesharrei, S., Rivas, J., Kucharski, F., Kirk-Davidoff, D., Bach, E., and Zeng, N.: Climate model shows large-scale wind and solar farms in the Sahara increase rain and vegetation, Science, 361, 1019–1022, https://doi.org/10.1126/science.aar5629, 2018.
Luo, N., Villar-Salvador, P., Li, G., and Wang, J.: The dark side of nursery photoperiod reduction on summer plantation performance of a temperate conifer: High winter mortality mediated by reduced seedling carbohydrate and nitrogen storage, Forest. Ecol. Manage., 491, 91–99, https://doi.org/10.1016/j.foreco.2021.119171, 2021.
Mitchard, E. T. A.: The tropical forest carbon cycle and climate change, Nature, 559, 527–534, https://doi.org/10.1038/s41586-018-0300-2, 2018.
Moore, J. C., Grinsted, A., Guo, X., Yu, X., Jevrejeva, S., Rinke, A., Cui, X., Kravitz, B., Lenton, A., Watanabe, S., and Ji, D.: Atlantic hurricane surge response to geoengineering, P. Natl. Acad. Sci. USA, 112, 13794–13799, https://doi.org/10.1073/pnas.1510530112, 2015.
Oren, R., Ellsworth, D. S., Johnsen, K. H., Phillips, N., Ewers, B. E., Maier, C., Schäfer, K. V. R., McCarthy, H., Hendrey, G., McNulty, S. G., and Katul, G. G.: Soil fertility limits carbon sequestration by forest ecosystems in a CO2-enriched atmosphere, Nature, 411, 469–472, https://doi.org/10.1038/35078064, 2001.
Pan, Y., Birdsey, R. A., Fang, J., Houghton, R., Kauppi, P. E., Kurz, W. A., Phillips, O. L., Shvidenko, A., Lewis, S. L., Canadell, J. G., Ciais, P., Jackson, R. B., Pacala, S. W., McGuire, A. D., Piao, S., Rautiainen, A., Sitch, S., and Hayes, D.: A large and persistent carbon sink in the world's forests, Science, 333, 988–993, https://doi.org/10.1126/science.1201609, 2011.
Pires, J. P. A., Marino, N. A. C., Silva, A. G., Rodrigues, P. J. F. P., and Freitas, L.: Tree community phenodynamics and its relationship with climatic conditions in a lowland tropical rainforest, Forests, 9, 1–14, https://doi.org/10.3390/f9030114, 2018.
Proctor, J., Hsiang, S., Burney, J., Burke, M., and Schlenker, W.: Estimating global agricultural effects of geoengineering using volcanic eruptions, Nature, 560, 480–483, https://doi.org/10.1038/s41586-018-0417-3, 2018.
Realmonte, G., Drouet, L., Gambhir, A., Glynn, J., Hawkes, A., Köberle, A. C., and Tavoni, M.: An inter-model assessment of the role of direct air capture in deep mitigation pathways, Nat. Commun., 10, 109–112, https://doi.org/10.1038/s41467-019-10842-5, 2019.
Reich, P. B., Sendall, K. M., Stefanski, A., Rich, R. L., Hobbie, S. E., and Montgomery, R. A.: Effects of climate warming on photosynthesis in boreal tree species depend on soil moisture, Nature, 562, 263–267, https://doi.org/10.1038/s41586-018-0582-4, 2018.
Riahi, K., van Vuuren, D. P., Kriegler, E., Edmonds, J., O'Neill, B. C., Fujimori, S., Bauer, N., Calvin, K., Dellink, R., Fricko, O., Lutz, W., Popp, A., Cuaresma, J. C., KC, S., Leimbach, M., Jiang, L., Kram, T., Rao, S., Emmerling, J., Ebi, K., Hasegawa, T., Havlik, P., Humpenöder, F., Da Silva, L. A., Smith, S., Stehfest, E., Bosetti, V., Eom, J., Gernaat, D., Masui, T., Rogelj, J., Strefler, J., Drouet, L., Krey, V., Luderer, G., Harmsen, M., Takahashi, K., Baumstark, L., Doelman, J. C., Kainuma, M., Klimont, Z., Marangoni, G., Lotze-Campen, H., Obersteiner, M., Tabeau, A., and Tavoni, M.: The Shared Socioeconomic Pathways and their energy, land use, and greenhouse gas emissions implications: An overview, Global Environ. Change, 42, 153–168, https://doi.org/10.1016/j.gloenvcha.2016.05.009, 2017.
Rivera, G., Elliott, S., Caldas, L. S., Nicolossi, G., Coradin, V. T., and Borchert, R.: Increasing day-length induces spring flushing of tropical dry forest trees in the absence of rain, Trees-Struct. Funct., 16, 445–456, https://doi.org/10.1007/s00468-002-0185-3, 2002.
Robock, A., Marquardt, A., Kravitz, B., and Stenchikov, G.: Benefits, risks, and costs of stratospheric geoengineering, Geophys. Res. Lett., 36, 581–597, https://doi.org/10.1029/2009GL039209, 2009.
Sellers, P.: Biophysical models of land surface processes, in: Climate System Modelling, edited by: Trenberth, K. E., Cambridge University Press, https://doi.org/10.1017/CBO9780511805530.026, 1992.
Stubblebine, W., Langenheim, J. H., and Lincoln, D.: Vegetative Response to Photoperiod in the Tropical Leguminous Tree Hymenaea courbaril L, Biotropica, 10, 18–19, https://doi.org/10.2307/2388100, 1978.
Sullivan, M. J. P., Lewis, S. L., Affum-Baffoe, K., Castilho, C., Costa, F., Sanchez, A. C., Ewango, C. E. N., Hubau, W., Marimon, B., Monteagudo-Mendoza, A., Qie, L., Sonké, B., Martinez, R. V., Baker, T. R., Brienen, R. J. W., Feldpausch, T. R., Galbraith, D., Gloor, M., Malhi, Y., Aiba, S. I., Alexiades, M. N., Almeida, E. C., De Oliveira, E. A., Dávila, E. Á., Loayza, P. A., Andrade, A., Vieira, S. A., Aragão, L. E. O. C., Araujo-Murakami, A., Arets, E. J. M. M., Arroyo, L., Ashton, P., Gerardo Aymard, C., Baccaro, F. B., Banin, L. F., Baraloto, C., Camargo, P. B., Barlow, J., Barroso, J., Bastin, J. F., Batterman, S. A., Beeckman, H., Begne, S. K., Bennett, A. C., Berenguer, E., Berry, N., Blanc, L., Boeckx, P., Bogaert, J., Bonal, D., Bongers, F., Bradford, M., Brearley, F. Q., Brncic, T., Brown, F., Burban, B., Camargo, J. L., Castro, W., Céron, C., Ribeiro, S. C., Moscoso, V. C., Chave, J., Chezeaux, E., Clark, C. J., De Souza, F. C., Collins, M., Comiskey, J. A., Valverde, F. C., Medina, M. C., Da Costa, L., Dančsák, M., Dargie, G. C., Davies, S., Cardozo, N. D., De Haulleville, T., De Medeiros, M. B., Del Aguila Pasquel, J., Derroire, G., Di Fiore, A., Doucet, J. L., Dourdain, A., Droissart, V., Duque, L. F., Ekoungoulou, R., Elias, F., Erwin, T., Esquivel-Muelbert, A., Fauset, S., Ferreira, J., Llampazo, G. F., Foli, E., Ford, A., Gilpin, M., Hall, J. S., Hamer, K. C., Hamilton, A. C., Harris, D. J., Hart, T. B., Hédl, R., Herault, B., Herrera, R., Higuchi, N., Hladik, A., Coronado, E., and Phillips, O. L.: Long-term thermal sensitivity of earth's tropical forests, Science, 368, 869–874, https://doi.org/10.1126/science.aaw7578, 2020.
Tokarska, K. B. and Zickfeld, K.: The effectiveness of net negative carbon dioxide emissions in reversing anthropogenic climate change, Environ. Res. Lett., 10, 64–70, https://doi.org/10.1088/1748-9326/10/9/094013, 2015.
Trenberth, K. E., Fasullo, J. T., and Kiehl, J.: Earth's global energy budget, B. Am. Meteorol. Soc., 90, 311–323, https://doi.org/10.1175/2008BAMS2634.1, 2009.