the Creative Commons Attribution 4.0 License.
the Creative Commons Attribution 4.0 License.
Global climate change and the Baltic Sea ecosystem: direct and indirect effects on species, communities and ecosystem functioning
Markku Viitasalo
Erik Bonsdorff
Climate change has multiple effects on Baltic Sea species, communities and ecosystem functioning through changes in physical and biogeochemical environmental characteristics of the sea. Associated indirect and secondary effects on species interactions, trophic dynamics and ecosystem function are expected to be significant. We review studies investigating species-, population- and ecosystem-level effects of abiotic factors that may change due to global climate change, such as temperature, salinity, oxygen, pH, nutrient levels, and the more indirect biogeochemical and food web processes, primarily based on peer-reviewed literature published since 2010.
For phytoplankton, clear symptoms of climate change, such as prolongation of the growing season, are evident and can be explained by the warming, but otherwise climate effects vary from species to species and area to area. Several modelling studies project a decrease of phytoplankton bloom in spring and an increase in cyanobacteria blooms in summer. The associated increase in N:P ratio may contribute to maintaining the “vicious circle of eutrophication”. However, uncertainties remain because some field studies claim that cyanobacteria have not increased and some experimental studies show that responses of cyanobacteria to temperature, salinity and pH vary from species to species. An increase of riverine dissolved organic matter (DOM) may also decrease primary production, but the relative importance of this process in different sea areas is not well known. Bacteria growth is favoured by increasing temperature and DOM, but complex effects in the microbial food web are probable. Warming of seawater in spring also speeds up zooplankton growth and shortens the time lag between phytoplankton and zooplankton peaks, which may lead to decreasing of phytoplankton in spring. In summer, a shift towards smaller-sized zooplankton and a decline of marine copepod species has been projected.
In deep benthic communities, continued eutrophication promotes high sedimentation and maintains good food conditions for zoobenthos. If nutrient abatement proceeds, improving oxygen conditions will first increase zoobenthos biomass, but the subsequent decrease of sedimenting matter will disrupt the pelagic–benthic coupling and lead to a decreased zoobenthos biomass. In the shallower photic systems, heatwaves may produce eutrophication-like effects, e.g. overgrowth of bladderwrack by epiphytes, due to a trophic cascade. If salinity also declines, marine species such as bladderwrack, eelgrass and blue mussel may decline. Freshwater vascular plants will be favoured but they cannot replace macroalgae on rocky substrates. Consequently invertebrates and fish benefiting from macroalgal belts may also suffer. Climate-induced changes in the environment also favour establishment of non-indigenous species, potentially affecting food web dynamics in the Baltic Sea.
As for fish, salinity decline and continuing of hypoxia is projected to keep cod stocks low, whereas the increasing temperature has been projected to favour sprat and certain coastal fish. Regime shifts and cascading effects have been observed in both pelagic and benthic systems as a result of several climatic and environmental effects acting synergistically.
Knowledge gaps include uncertainties in projecting the future salinity level, as well as stratification and potential rate of internal loading, under different climate forcings. This weakens our ability to project how pelagic productivity, fish populations and macroalgal communities may change in the future. The 3D ecosystem models, food web models and 2D species distribution models would benefit from integration, but progress is slowed down by scale problems and inability of models to consider the complex interactions between species. Experimental work should be better integrated into empirical and modelling studies of food web dynamics to get a more comprehensive view of the responses of the pelagic and benthic systems to climate change, from bacteria to fish. In addition, to better understand the effects of climate change on the biodiversity of the Baltic Sea, more emphasis should be placed on studies of shallow photic environments.
The fate of the Baltic Sea ecosystem will depend on various intertwined environmental factors and on development of the society. Climate change will probably delay the effects of nutrient abatement and tend to keep the ecosystem in its “novel” state. However, several modelling studies conclude that nutrient reductions will be a stronger driver for ecosystem functioning of the Baltic Sea than climate change. Such studies highlight the importance of studying the Baltic Sea as an interlinked socio-ecological system.
Global climate change affects the marine ecosystem through ocean warming, acidification and deoxygenation and through changes in nutrient loading and water circulation, which may all impact marine biological processes from genes to populations, communities and ecosystems (Brierley and Kingsford, 2009; Henson et al., 2017). The biological consequences range from shifts in species abundance and distributions, changes in dispersal patterns and modification of species interactions to altered food webs and decreasing ocean productivity (Hoegh-Guldberg and Bruno, 2010; Philippart et al., 2011; Doney et al., 2012; Burrows et al., 2019). The changes in biological processes also affect marine ecosystem services and threaten human food security, especially in the most vulnerable areas (Barange et al., 2014).
Climate change also has multiple effects on the Baltic Sea, impacting species, communities and ecosystem functioning. As in the ocean, the effects are usually mediated via climate-affected oceanographic or biogeochemical processes and via associated indirect effects on species interactions, trophic dynamics and ecosystem function mechanisms. These potentially affect the biota inhabiting the Baltic Sea, as well as the human society (Paasche et al., 2015; Hyytiäinen et al., 2019; Pihlainen et al., 2020; Stenseth et al., 2020).
The effects of climate change on the Baltic Sea ecosystem may differ from those projected for the oceanic areas, as the Baltic Sea differs in many respects from the oceans and even from the coastal ecosystems surrounding the other regional seas and oceans. The communities of the Baltic Sea are formed of a peculiar combination of marine, limnetic and brackish-water taxa. The long winter and the strong seasonal cycle give the area subarctic properties, especially in the northern areas. The Baltic Sea has also been shown to warm up faster than most other sea areas of the world (Belkin, 2009; Sherman et al., 2009), albeit with large differences between sub-basins (Kniebusch et al., 2019; Dutheil et al., 2021).The Baltic Sea is also strongly affected by its watershed, which is more than 4 times larger than its surface area and is inhabited by ca. 85 million people (Omran and Negm, 2020). The marine ecosystem therefore receives excess nutrients and other elements and contaminants from the land via rivers, through the air, and by leaking from the sediments of the Baltic Sea. Furthermore, the irregular inflows of more saline and oxic North Sea water, which at specific basin-wide weather conditions enter the Baltic sea through the Danish Straits (Matthäus and Schinke, 1994; Lehmann et al., 2022) and influence the state and functioning of the Baltic Sea.
All of these pathways of chemical elements and oceanographic and biogeochemical processes may be affected by global climate change and the quasi-cyclic climate phenomena such as the North Atlantic Oscillation (NAO). It has also been suggested that impacts and symptoms of global climate change are accumulating faster in the Baltic Sea than in other coastal areas of the oceans and that Baltic Sea thus can be considered as “a time machine for the future coastal ocean” (Reusch et al., 2018).
However, attribution of the observed ecosystem changes to global (anthropogenic) climate change is challenging because of the multiple synergistic effects between climate and other environmental drivers, such as eutrophication, harmful substances, habitat modification, fishing and introduction of non-indigenous species, which all may have strong impacts on ecosystems and their functioning in time and space (Reusch et al., 2018; Stenseth et al., 2020; Bonsdorff, 2021). Therefore, profound knowledge of the mechanisms and processes governing the Baltic Sea ecosystem under climate change are vital for the understanding and management of the Baltic Sea (Reusch et al., 2018; Bonsdorff, 2021; Blenckner et al., 2021).
The overall effects of climate change on the Baltic Sea have been reviewed in earlier synthesis studies (The BACC Author Team, 2008; The BACC II Author Team, 2015), in which climate impacts on the marine ecosystem were also assessed (Dippner et al., 2008; Viitasalo et al., 2015). Since then, a wealth of field, experimental and modelling studies have shed more light onto the complex interactions between the climate change and the Baltic Sea system (Meier et al., 2022b).
In this paper, we review research on climate change effects on the Baltic Sea species, habitats, and ecosystem functioning, primarily based on research published in 2010–2021. We include both studies investigating direct effects of climate-related parameters on organisms, as well as studies that investigate the more indirect processes affecting the structure and functioning of the Baltic Sea ecosystem through biogeochemistry and food web interactions. Evidence is compiled from empirical field studies that show past changes and responses of species, populations, and communities to climate-affected parameters such as temperature, salinity, oxygen and pH. A large number of experimental studies, investigating species responses to the same parameters in microcosms or mesocosms, are reviewed. Studies investigating the complex effects of climate change on the interactions between species and trophic groups, i.e. phytoplankton, bacteria, cyanobacteria, zooplankton and fish, as well as algae or vascular plants and invertebrates grazing on them, are also analysed. Modelling studies, based on coupled oceanographic–biogeochemical models or other types of species-level or food web models, are reviewed. Based on the published research we draw conclusions about the role of climate-driven environmental variables on shaping the structure and functioning of the Baltic Sea ecosystem and identify knowledge gaps and current issues of dissensus. Areas in need of more research are recommended.
We review studies that shed light to the possible climate effects on the Baltic Sea ecosystem by studying oceanographic and biogeochemical parameters which have been projected to change due to climate change. As such changes may be affected by both anthropogenic global climate change and natural climate variations, it is first necessary to define certain key terms used in this review.
By “global climate change” we refer to the past and contemporary increase in global temperature caused by anthropogenic emissions of CO2 and other greenhouse gases and its effects on various climatic, oceanographic and biogeochemical parameters. By “climate change”, in turn, we refer to a large-scale shift in climatic parameters affecting the Baltic Sea region that may be caused either by global climate change, by cyclic climate fluctuations (such as the North Atlantic Oscillation, NAO), or by irregular or stochastic variation in climate parameters. We are not considering short-term (between-year or seasonal) weather variations but mainly include studies that attempt to reveal organisms' responses to longer-term (several years to decades) variability in climate.
For “ecosystem functioning” we use Tilman's (2001) definition, “the rate, level, or temporal dynamics of one or more ecosystem processes such as primary production, total plant biomass, or nutrient gain, loss, or concentration”. By “functional diversity” we mean “the range and value of those species and organismal traits that influence ecosystem functioning” (Tilman, 2001). A “functional group”, is “a set of species that have similar traits and that thus are likely to be similar in their effects on ecosystem functioning” (Tilman, 2001).
With “biogeochemical processes”, we refer to various biogeochemical cycles and processes, which often involve cycling and transfer of allochthonous or autochthonous essential nutrients and/or minerals and organic carbon and which are either driven or influence biological activity in species. With “trophic dynamics” we refer to interactions between trophic levels or functional groups, such as phytoplankton, bacteria, cyanobacteria, nanoflagellates and microflagellates, microzooplankton and mesozooplankton, zoobenthos, and fish, as well as the algae, vascular plants and invertebrates living amongst them.
“Trophic efficiency” is defined as “the efficiency of energy flow between trophic levels and is the percentage of energy from a trophic level that is used by the organisms of the next trophic level for growth and reproduction” (Hine, 2019).
The search for relevant papers was implemented mainly using Web of Science (WoS) website tool (https://apps.webofknowledge.com/, last access: 2 March 2022) maintained by Clarivate. The search was focused on years 2010–2021 and was performed using several search terms in various combinations. These included (always) “Baltic Sea” and (in various combinations) “climate”, “climate change”, “global climate change”, “marine ecosystem”, “temperature”, “salinity”, “acidification” and “pH”, as well as taxonomic groups such as “phytoplankton”, “cyanobacteria”, “bacteria”, “zooplankton”, “microzooplankton”, “mesozooplankton”, “flagellates”, “macroalgae”, “zoobenthos”, “benthic animals”, “fish”, and “microbial loop”. Marine birds and mammals were not included. The words were used as both title and topic search terms, and several reference lists were derived and merged.
Some papers from 2021 and 2022 were found and downloaded with an unstructured search performed with Google Scholar, as this website tool includes more recent publications than WoS. In some cases, references before 2010 were also included if it was necessary to back up the statements with older studies.
The search resulted in over 500 papers, of which many were not relevant to the current review, i.e. were not concerning effects of climate change on species, habitats or ecosystem functioning in the Baltic Sea. The most relevant studies were saved into library groups of EndNote X9.2 reference management software (Clarivate Analytics), and the contents were scrutinised in more detail.
Because of the focus period, 2010–2021, the review is not a full systematic review of all research done on climate change effects on the Baltic Sea ecosystem this far. Also, certain taxonomic groups and study types were less thoroughly reviewed than others. Fish studies in particular were not comprehensively scrutinised because the complex responses of fish populations to climate, eutrophication and fisheries have recently been addressed by a large number of studies and would merit their own review. Also, we have not reviewed all experimental studies that have dealt with environmental variables that may change with climate change. Our goal is to highlight the variety of field, experimental and modelling studies and to summarise what can be concluded from the recent evidence on the possible effects of climate change on the Baltic Sea.
4.1 Phytoplankton
Climate change may have direct effects on the physiology and phenology on phytoplankton through physical and chemical parameters and indirectly through hydrodynamics, e.g. stratification and availability of light and nutrients. Top-down forces, i.e. grazing on phytoplankton, may also be modified in various ways if grazer populations change.
The growing season of phytoplankton has been significantly prolonged with warming temperatures during the recent decades. A satellite-based study suggested that the length of the period with chlorophyll concentration of at least 3 mg m−3 has in the Baltic Sea doubled, from 110 d in 1998 to 220 d in 2013 (Kahru et al., 2016). Another study using phytoplankton sampling data from the Bay of Mecklenburg, in the western Baltic Sea, confirmed that the phytoplankton growing season, which in 1988–1992 on average lasted from March to August, now (2014–2017) extends from February to December (Wasmund et al., 2019), with a longer gap between the spring and late summer peaks. This prolongation was tentatively explained by increased sunshine in spring and higher temperature in the autumn, inducing changes in species composition and settling rates of phytoplankton, remineralisation of organic matter by bacteria, and grazing rates by zooplankton (Wasmund et al., 2019).
The spring species communities have also shifted from dominance of early blooming diatoms to later blooming dinoflagellates and the mixotrophic ciliate Mesodinium rubrum (Klais et al., 2011; Hällfors et al., 2013; Kuosa et al., 2017; Hjerne et al., 2019), probably due to changes in climate and weather patterns, including ice cover, solar irradiation and wind conditions (Klais et al., 2013; Hjerne et al., 2019). In the long-term data, variable results can be seen according to area and dominating species group. For instance, spring phytoplankton biomass increased in the Baltic Proper but decreased in the Belt Sea area (1979–2005); both areas showed antagonism between communities dominated by diatoms or dinoflagellates, and the trends were therefore oscillating rather than linear (Wasmund et al., 2011). Symptoms of a regime shift were identified, and changes were attributed to approximately 10-year fluctuations in temperature, salinity and nutrients. A linkage to global climate change was not detected (Wasmund et al., 2011).
Some studies have attributed the springtime shifts in phytoplankton phenology and community structure to changes in environmental conditions driven by global climate change. A 15-year study (2000–2014) using FerryBox observations, covering the area between Helsinki (Gulf of Finland) and Travemünde (Mecklenburg Bight), confirmed that spring bloom intensity was mainly determined by winter nutrient concentration, while bloom timing and duration co-varied with meteorological conditions. The authors conclude that the bloom magnitude has been affected by the reduction of nutrient loading from land, while bloom phenology can also be modified by global climate change affecting seasonal oceanographic and biogeochemical processes (Groetsch et al., 2016).
It has also been suggested that in the future climate higher temperatures and less ice will cause an earlier bloom of both diatoms and dinoflagellates, with increased dinoflagellate dominance (Hjerne et al., 2019). Experimental (mesocosm) evidence supports findings that warming up of water and changes in light conditions will accelerate the spring bloom, induce a decline in peak biomass and favour small size cells, either directly or via increased grazing by copepods (Sommer et al., 2012). On the other hand, this development may be counteracted by increases of windiness and cloudiness, which have also been projected by certain modelling studies (Hjerne et al., 2019). Recent studies have however indicated that the projections for spring and summer wind and radiation are uncertain (Christensen et al., 2022), and future weather changes and associated spring bloom dynamics therefore remain obscure.
Climate change effects, i.e. temperature increase, salinity decline and acidification have been shown to have variable results on the toxic dinoflagellate Alexandrium ostenfeldii. Generally, the growth rates, as well as saxitoxin production, increased with higher temperature and elevated pCO2, but the responses were variable between strains (Kremp et al., 2012, 2016). In contrast, temperature or salinity changes did not have a significant effect on cyst germination of this species (Jerney et al., 2019).
Climate change also increases concentration of water carbon dioxide, a compound necessary for primary production, and ocean acidification (OA) could therefore enhance productivity of phytoplankton. However, the results of experimental studies investigating effects of pCO2 on phytoplankton are variable. The biomass of southern Baltic autumn phytoplankton (kept in 1400 L indoor mesocosms for 21 d) increased when pCO2 was increased from 439 to 1040 ppm, also under warm conditions (Sommer et al., 2015). In other experiments, OA had little effect on community composition, fatty acid composition or biovolumes of phytoplankton in spring or autumn (Paul et al., 2015; Bermudez et al., 2016; Olofsson et al., 2019). Even when (positive) effects were detected, they were mainly caused by an associated decrease in grazing by copepod nauplii in low temperature treatments (Paul et al., 2016).
There are also studies that have indicated a connection between phytoplankton and the North Atlantic Oscillation (NAO). A decline in the intensity of NAO in the 1990s was suggested to have been caused by less cloudy conditions, giving more irradiance and less windy conditions, inducing stronger stratification of surface water (Hjerne et al., 2019). If the shifts are driven by variations in NAO or the Baltic Sea Index (BSI, a regional index similar to the NAO), they may be temporary and reversible, whereas shifts caused by global climate change may be more enduring.
In the northern Baltic Proper, Åland Sea and the Gulf of Finland, the biomasses of Chrysophyceae, Prymnesiophyceae and Cyanophyceae have increased and the phytoplankton biomass maximum, which in the 1980s was in spring and mainly consisted of diatoms, is now in July–August and is dominated by filamentous cyanobacteria (Suikkanen et al., 2013). This shift was explained by a complex interaction between eutrophication, climate-induced warming, and increased top-down pressure, as well as changes in DIN:DIP ratio in summer (Suikkanen et al., 2013). In the Gulf of Bothnia, a gradual decline in salinity was also an important factor for phytoplankton community change in 1979 to 2012 (Kuosa et al., 2017).
It is obvious that climatic influences are intertwined with other processes and parameters affecting phytoplankton, especially anthropogenic nutrient loading from land and internal loading of nutrients from the sediments. There is however a discrepancy in the relative effects of eutrophication, climate change, and other environmental and anthropogenic factors in explaining past variations in phytoplankton communities and biomass. Also, several studies have identified complex variations in phytoplankton communities that cannot be easily explained by any of the studied factors or environmental parameters.
A study comparing historic phytoplankton communities from 1903–1911 with the present ones (1993–2005) in the northern Baltic Proper and the Gulf of Finland observed an undefined “period effect”, characterised by a decline in diatoms and increase in dinoflagellates, that was not explained well by the available environmental variables (temperature, salinity, and general regional climatological data). Although data on biogeochemical parameters was not available for the period 1903–1911, the authors interpreted the observed community change as evidence of the direct and/or indirect influence of eutrophication (Hällfors et al., 2013).
A study investigating summer phytoplankton time series (HELCOM monitoring 1979–2012) across the Baltic Sea found that there were no common interannual patterns. Instead, the class trends, e.g. that of cryptophytes, may be affected by anomalies in the BSI, although a mechanistic explanation for the relationship could not be found (Griffiths et al., 2020). Other studies did not find any explanation for the observed changes in the biovolumes of different taxa, e.g. decrease in diatoms and increase in certain dinoflagellate taxa, and concluded that phytoplankton community in the Baltic Sea is not in a steady state (Olli et al., 2011), or noted that stochastic dynamics at local scales confound any commonalities between phytoplankton groups (Griffiths et al., 2020).
To sum up, the past changes in phytoplankton community composition have been very variable and usually cannot be explained by a single factor. Some clear signs of climate change, such as prolongation of the growing season are evident, and can be explained by the warming and associated biogeochemical processes, but the changes in species and communities vary from area to area and have multiple reasons, including climate change, changes in nutrient dynamics and changes in trophic interactions.
4.2 Cyanobacteria
Filamentous diazotrophic cyanobacteria benefit from warm temperatures and stratified water, and they tend to bloom during the hottest and calmest periods of summer (Munkes et al., 2021). Several modelling studies suggest that the climate-induced increase in stratification (Liblik and Lips, 2019), together with potentially increasing hypoxia and consequent release of phosphorus from the anoxic sediments, will increase cyanobacteria blooms in the Baltic Sea (Meier et al., 2011a; Neumann et al., 2012; Chust et al., 2014; Lessin et al., 2014; Andersson et al., 2015; Ryabchenko et al., 2016).
Many field studies have also stated that cyanobacteria have already increased along with the warming of the Baltic Sea. In the northern Baltic Proper, Åland Sea and the Gulf of Finland, the biomasses of Cyanophyceae have increased, which has been explained by an interaction between warming, eutrophication and increased top-down pressure on species of the spring bloom, as well as changes in DIN:DIP ratio in summer (Suikkanen et al., 2013).
Also, in the Gulf of Bothnia, eutrophication and cyanobacteria have increased in summer (Fleming-Lehtinen et al., 2015; Kuosa et al., 2017), and extensive cyanobacteria blooms have in the past few years been detected with satellite methods in the Bothnian Sea, an area usually devoid of such phenomena (unpublished monitoring and satellite records collected by the Finnish Environment Institute). The increase of cyanobacteria in the Bothnian Sea has been attributed to an increased freshwater flow and, since 2000, to an increased intrusion of more saline and phosphorus rich Baltic Proper water into the Bothnian Sea. These changes have increased stratification, lowered oxygen conditions, and led to a decline in N:P ratio of the Bothnian Sea, which has favoured the development of cyanobacteria blooms in the area (Rolff and Elfwing, 2015; Ahlgren et al., 2017; Kuosa et al., 2017)
It has also been suggested that the various drivers of climate change may contribute to increase blooms and toxicity of cyanobacteria in the Baltic Sea. For instance, the intracellular toxin concentration of the cyanobacterium Dolichospermum sp. may increase with elevated temperature (+4 ∘C) (Brutemark et al., 2015; Wulff et al., 2018) and with decreased salinity (from 6 to 3) (Wulff et al., 2018). As toxins of both dinoflagellates (Sopanen et al., 2011) and cyanobacteria (Karjalainen et al., 2006, 2007; Engström-Öst et al., 2017) can accumulate in Baltic Sea zooplankton and induce lower grazing rates and higher mortality, these studies suggest that toxic dinoflagellates and filamentous cyanobacteria may get a competitive advantage over diatoms and other phytoplankton in a future Baltic Sea due to their toxic effects and unpalatability.
A few long-term studies have not found an increase in cyanobacteria during the past. Two recent studies compiling monitoring data from the Baltic Sea for 1979–2012 (Griffiths et al., 2020) and 1979–2017 (Olofsson et al., 2020) did not find any evidence for an overall increase of diazotrophic filamentous cyanobacteria during this period. Biovolume of the hepatotoxic Nodularia spumigena did not change, and that of the non-toxic Aphanizomenon sp. increased in the north and declined in the south (Olofsson et al., 2020). Also, a study that compared the years 1903–1911 and 1993–2005 concluded that cyanophyte biomass has not increased in summer and has decreased in spring and autumn (Hällfors et al., 2013). It has been suggested that although cyanobacteria do prefer warmer temperatures, the effect of ongoing warming can better be seen in changes in phenology of cyanobacteria rather than as an increase of biomass (Griffiths et al., 2020). Also, a connection between the amount of cyanobacteria and the Baltic Sea Index has been identified (Griffiths et al., 2020).
Hypothetically, ocean acidification could benefit cyanobacteria through increased availability of carbon dioxide in water. The available studies do not give a definitive answer however. When pCO2 was experimentally increased, the production of single-celled cyanobacterium Cyanothece increased, while that of Nodularia sp. decreased (Eichner et al., 2014). Also, an increase in temperature from 16 to 18–20 ∘C led to an earlier peak of cyanobacteria, while the biomass of cyanobacteria, especially that of nitrogen-fixer Dolichospermum sp. declined (Berner et al., 2018). Further, in mesocosm studies an increase of pCO2 (from 360 to 2030 µatm) coupled with an increase in water temperature (from 16.6 to 22.4 ∘C) had a negative impact on the biomass of the diazotrophic cyanobacteria Nodularia spumigena (in 1400 L mesocosms, 28 d) (Paul et al., 2018). Another experimental study (using 75 mL cell culture flasks), investigating the effects of increased temperature (from 12 to 16 ∘C), decreased salinity (from 7 to 4) and elevated pCO2 (from 380 to 960 ppm), found that only temperature had an effect on biovolume and photosynthetic activity of Nodularia spumigena and Aphanizomenon sp. (Karlberg and Wulff, 2013). The two species however had antagonistic effects on each other: biovolumes were lower when grown together than when grown separately, indicating species interactions.
If the biomasses of Nodularia sp. and Dolichospermum decrease due to increased acidification, nitrogen input into the Baltic Sea and carbon export to heterotrophic bacteria via cyanobacteria might decline (Eichner et al., 2014; Berner et al., 2018). This could however be balanced by the potential increase of Cyanothece, which is also a nitrogen fixer (Eichner et al., 2014).
To sum up, there are species-specific responses to climate change and associated oceanographic parameters within cyanobacteria. Several field and modelling studies suggest that the climate-induced increase in temperature and stratification, together with increasing hypoxia and release of phosphorus from the sediments, has increased cyanobacteria biomass and will also continue to favour cyanobacteria blooms in the future. However, the results of certain empirical and experimental studies give a more multifaceted picture of cyanobacteria response to climate change. The past increase in cyanobacteria is not as obvious as might be expected, responses vary from species to species, and processes affecting the amount of cyanobacteria in the Baltic Sea can be modified, counteracted or amplified by various environmental processes and food web interactions.
4.3 Mesozooplankton
The Baltic Sea mesozooplankton species originate either from marine or freshwater environments, and some are typically found in brackish water. It is therefore plausible that they respond to long-term variations in oceanographic parameters. Several field studies have confirmed that marine copepod species (e.g., Pseudocalanus spp. and Temora longicornis) declined during the 1980s and 1990s, while smaller-sized euryhaline and limnetic copepod species (Acartia spp. and Eurytemora spp.) increased in abundance (Suikkanen et al., 2013; Hänninen et al., 2015), and the decline of marine taxa has usually been proposed to be linked to a decrease of salinity (Suikkanen et al., 2013; Hänninen et al., 2015). It has also been experimentally shown that respiration of copepods (Acartia longiremis) increases and feeding rate decreases (in 610 mL bottles, 24 h experiments) close to the physiological tolerance limit for salinity (below 7 psu), indicating a disruption of the energetic balance under low salinity (Dutz and Christensen, 2018).
Environmental impacts on the physiology of the more sensitive species may also affect the reproductive success of zooplankton (Möller et al., 2015). The increase of euryhaline taxa has been directly or indirectly attributed to the temperature increase (Mäkinen et al., 2017). It has also been suggested that species that reside in the upper water layers, such as the copepod Acartia sp., are mostly affected by temperature-driven increase in food availability, whereas species inhabiting the deep layers, such as older stages of Pseudocalanus acuspes, are more dependent on salinity and predation pressure (Otto et al., 2014a, b; Mäkinen et al., 2017).
The effects of climate-driven variations in temperature and ocean acidification (OA) on zooplankton have been studied experimentally. In Acartia sp., warming decreased egg viability, nauplii development and adult survival (in 1.2 L bottles, during 60 h) (Vehmaa et al., 2013). In other experiments, both warming (Garzke et al., 2015) and OA (Vehmaa et al., 2016) had negative effects on adult female size. This suggests that the projected warming combined with ocean acidification may have negative effects on the populations of these copepods in the future Baltic Sea.
Changes in zooplankton functional groups, such as a shift from raptorial and suspension-feeding copepods and cladocerans to a dominance of small filter-feeding rotifers and cladocerans, have also been shown as results of warming (Suikkanen et al., 2013; Jansson et al., 2020). OA also promoted the growth of suspension-feeding cladocerans because of a CO2-driven increase in cyanobacteria (Lischka et al., 2017).
Furthermore, a switch from predominantly herbivorous feeding by copepods to predation on ciliates has been observed in a field study in the southern and central Baltic Sea during cyanobacterial blooms (Loick-Wilde et al., 2019). This was caused by decomposing of the otherwise unpalatable filamentous cyanobacteria and an associated increase of the bacteria, nanoflagellates and ciliates (Hogfors et al., 2014). Warming may also increase zooplankton grazing on medium to large algae, which could contribute to a change towards smaller-sized phytoplankton species (Klauschies et al., 2012; Paul et al., 2015). It is therefore possible that the dominant traits of zooplankton communities will change if climate-induced warming and reduced salinity trends continue. It has also been suggested, from experimental (mesocosm) evidence, that warming speeds up the growth of copepods but leaves phytoplankton unaffected, which shortens the time lag between phytoplankton and zooplankton. This may lead to a larger and earlier zooplankton peak and increase the possibility of zooplankton controlling phytoplankton, which may lead to a reduced phytoplankton biomass under warm temperature (Paul et al., 2016).
Sufficient supply of essential compounds such as amino acids (AA) produced by phytoplankton and cyanobacteria is essential for the growth and productivity of zooplankton grazers. A field study performed in the Baltic Proper shows that, during a warm summer, thermophilic rotifers and cladocerans (e.g. Bosmina spp.) acquired ample AA through filter feeding on the abundant diazotrophic cyanobacteria, whereas the temperate copepods (e.g. copepods Temora longicornis and Pseudocalanus spp.) avoided the warm surface layer and acquired AA mainly through sinking organic matter and/or via grazing on chemoautotroph-based microbial food web in the suboxic zone (Eglite et al., 2018). Mesocosm experiments have also demonstrated that a high bacterial production can maintain copepod production (Lefébure et al., 2013) but that increased heterotrophy leads to a decreased fatty acid content and lower individual weight of copepods (Dahlgren et al., 2011). This may imply that thermophilic zooplankton species, such as rotifers and certain cladocerans gain more AA than copepods in a future warmer Baltic Sea.
Little is known about the adaptation capabilities of zooplankton against physicochemical stress, but some degree of temperature adaptation has been demonstrated experimentally for the copepod Eurytemora affinis (Karlsson and Winder, 2020). Interestingly, the adaptability was better in populations reared in warm temperatures (≥17 ∘C), which suggests that southern populations can better cope with increasing temperatures than the northern ones and that the adaptation capability of all (surviving) populations may improve with proceeding climate change.
To sum up, a shift towards smaller-sized zooplankton and a stronger linkage between mesozooplankton and the microbial food web is probable in a warmer Baltic Sea. A decline in certain marine species has also been projected, but this will depend on the future velocity of salinity decline, patterns of stratification, realised time lag between phytoplankton and zooplankton peaks, predation pressure by fish, and the possible adaptation of zooplankton species to the subtle changes in salinity.
4.4 Bacteria and the microbial food web
Bacteria are key components of the ecosystem, as they decompose organic material and serve as food for heterotrophic nanoflagellates and the associated microbial food web. They affect the nutrient and carbon dynamics of the marine ecosystem, and it is therefore possible that climate impacts on bacteria may radiate to the structure and functioning of the entire Baltic Sea ecosystem.
The effects of climate-induced changes in environmental factors to pelagic bacteria and the other components of the microbial food web have been studied experimentally. The effects of projected ocean acidification (OA) on bacteria have been studied alone and also in combination with other abiotic variables, such as temperature (OAW) and salinity (OAS). OA alone had a limited impact on spring bloom microbial communities (sampled from the sea area around the island Öland in the Baltic Proper and kept in 100 L mesocosms for 21 d), but when combined with increased temperature, certain bacterial phylotypes, such as betaproteobacteria, increased. It was suggested that synergistic effects of increased temperature and acidification selectively promote growth of specific bacterial populations (Lindh et al., 2013). In the southern Baltic Sea (Kiel Bight) the impact of OA was studied in 1400 L indoor mesocosms for 21–24 d. Acidification only affected a few operational taxonomic units (OTUs), such as Bacteroidetes “NS3a marine group”, as the bacterial community mainly responded to temperature and phytoplankton succession. Depending on studied season and temperature treatment, Cyanobacteria, Bacteroidetes, Alphaproteobacteria and/or Gammaproteobacteria increased under OA (Bergen et al., 2016).
In an OAS experiment (4 L aquaria, 12 d) using a natural summer microplanktonic community, the biovolume of heterotrophic bacteria declined when pCO2 was increased (from 380 to 960 µatm) and salinity was decreased (from 6 to 3 psu) (Wulff et al., 2018). In experiments done in the Baltic Proper (NW Gotland Sea, 25 L microcosms for 23 d), where temperature was increased (from 16 to 18–20 ∘C) and salinity reduced (from 6.9 to 5.9 PSU), the microbial community showed mixed responses. No conclusive evidence of direct climate-induced change could be detected (Berner et al., 2018). At reduced salinity levels, certain Actinobacteria and Bacteroidetes OTUs increased, and the heterotrophic bacteria community resembled communities at high temperature, indicating synergistic effects of temperature and salinity. Biotic interactions were more dominant than abiotic ones however. The largest increase in heterotrophic bacterial biomass was detected when filamentous cyanobacteria started to decay, regardless of temperature or salinity. It was suggested that this indirect coupling between heterotrophic bacteria and filamentous cyanobacteria is more important for bacterial communities than the direct effects of climate-induced changes in temperature or salinity (Berner et al., 2018).
Experimental studies have demonstrated that complex food web responses to climate change may also arise. In the Quark, located in the Gulf of Bothnia, an increase in dissolved organic matter (DOM) enhanced respiration and abundance of bacteria, whereas an increase in temperature (from 12 to 15 ∘C) induced a decrease in bacteria, probably due to an increase in bacterivorous flagellates (Nydahl et al., 2013). A complex response to warming was also demonstrated for different size classes of heterotrophic flagellates (HFs). There was a succession from flagellates feeding on bacteria to omnivorous nanoflagellates preying upon other HF. This intraguild predation pattern probably dampened the response to experimental treatments (Moustaka-Gouni et al., 2016). Mesocosm experiments done in the Gulf of Bothnia area have however demonstrated that increasing dissolved organic carbon (DOC) enhances bacterial production and leads to a promotion of heterotrophy (Dahlgren et al., 2011; Andersson et al., 2013). Mixing depth also influences the ratio of heterotrophic to autotrophic production: with a shallow pycnocline, the autumn plankton community in the northern Bothnian Sea remained net autotrophic irrespective of DOC, whereas with increased mixing depth and with added DOC the system became net heterotrophic (Båmstedt and Wikner, 2016).
As for microzooplankton (MZP), the effects of OA and warming seem to be mostly beneficial. OA does not have a negative effect on MZP, probably because estuarine MZP are adapted to a large natural variability in pCO2 (Horn et al., 2016). The abundance of the mixotrophic ciliate Mesodinium sp. even increased in mesocosms with OA because of increase of its prey and food, e.g. picoeukaryotes, at higher CO2 levels (Lischka et al., 2017). In addition, warming improved the growth rate of MZP, and their biomass peaked earlier in warm mesocosm treatments. This led to a reduced time lag between MZP and phytoplankton peaks, inducing a better food supply to microzooplankton in warm conditions (Horn et al., 2016). The same applied to the MZP-copepod link: at low temperatures MZP escaped from predation by slower growing copepods, whereas at higher temperatures especially small-sized ciliates were more strongly controlled by copepod predation.
To sum up, different components of the microbial food web show very variable responses to climate-induced changes in temperature, salinity and pH. Bacteria growth is generally favoured by increasing temperature, but mixed effects are common, and indirect processes affecting decay and availability of organic matter and abundances of species predating on bacteria are also important. This highlights the importance of considering the effects of abiotic factors and the delicate indirect food web effects on the dynamics of the microbial food web and the pelagic ecosystem in general.
4.5 Macroalgae and vascular plants
Long-term changes in Baltic Sea macroalgae and charophytes have mostly been explained by combined or synergistic effects of changes in salinity, wind exposure, nutrient availability and water transparency (Gubelit, 2015; Blindow et al., 2016; Eveleens Maarse et al., 2020; Rinne and Salovius-Laurén, 2020) and by biotic interactions (Korpinen et al., 2007).
For the brown alga bladderwrack Fucus spp., light availability, which may also be affected by partly climate-driven changes in eutrophication, affects their local coverage (Lappalainen et al., 2019). A documented long-term decrease in water transparency in 1936 to 2017 has reduced favourable sea floor areas for Fucus spp. by 45 % (Sahla et al., 2020), and resulted in a halving of the depth range of F. vesiculosus in the Åland Islands (Eveleens Maarse et al., 2020). For many shallow coastal ecosystems of the Baltic Sea, it has been concluded that eutrophication is the most important pressure affecting the ecosystem structure and functioning (Olsson et al., 2015). This is plausible because of the strong influence of anthropogenic nutrient loading in coastal areas (Vigouroux et al., 2021), especially those that are prone to hypoxia due to complex topography (Virtanen et al., 2019), and which often are affected by internal loading of phosphorus from the sediment (Puttonen et al., 2014, 2016). The effects of anthropogenic eutrophication on macroalgae may however be amplified or counteracted by climate-induced changes in environmental parameters. Such interactions are reviewed below.
The direct effects of climate-induced changes in temperature, salinity and ocean acidification (OA) on bladderwrack Fucus vesiculosus have been investigated by a number of experimental studies. OA appears to have a relatively small effect on macroalgae (Al-Janabi et al., 2016a; Wahl et al., 2019), while temperature effects may be significant. The impacts of increasing temperature are not linear however. Growth or photosynthesis is not impaired under temperatures of 15 to 17.5 ∘C but at extreme temperatures, simulating heatwaves of 27 to 29 ∘C, photosynthesis declines, growth ceases and necrosis starts (Graiff et al., 2015; Takolander et al., 2017b). Necrosis is also enhanced by low salinity (4 PSU) (Takolander et al., 2017b), and under very low salinity (2.5 PSU) the sexual reproduction of F. vesiculosus ceases (Rothäusler et al., 2018, 2019).
The timing of temperature stress is however important for the damage experienced by algae. Experiments done with bladderwrack Fucus vesiculosus (in 1500 L tanks in Kiel, in the western Baltic Sea) showed that in the early and late summer warming of 1 to 5 ∘C above ambient had mostly beneficial effects on bladderwrack, whereas in midsummer a similar warming had negative effects (Wahl et al., 2021). During midsummer, the heatwaves surpassed the physiological tolerance limits of the species, with detrimental effects on growth.
Ocean acidification combined with warming (OAW) may also act in concert with hypoxia in areas where upwellings bring hypoxic water close to the surface. In a 3 d experiment simulating an upwelling event, hypoxic water caused severe mortality of Fucus germlings if they were already stressed by OAW (Al-Janabi et al., 2016b).
Climate-induced decline in salinity may affect communities via its direct effect on the physiology of individual populations and species. A retreat towards the south–south-west has been predicted for marine species such as bladderwrack and eelgrass and for species affiliated to them (Vuorinen et al., 2015). Species distribution modelling studies have suggested that this mainly salinity-induced decrease of bladderwrack will cause habitat fragmentation with large effects on the biodiversity and ecosystem functioning of the shallow water communities of the northern Baltic Sea (Takolander et al., 2017a; Jonsson et al., 2018; Kotta et al., 2019).
It is not certain to what degree Fucus vesiculosus can adapt to the anticipated changes. It has been suggested that Baltic marine species have, due to local adaptation, isolation and genetic endemism, diminished potential for adaptation, and they therefore have an increased risk of local extinction (Johannesson et al., 2011). For example, F. vesiculosus has a long generation time and relatively restricted dispersal, and therefore the dispersal rate of locally adapted genotypes may not keep pace with the projected velocity of salinity decline (Jonsson et al., 2018). However, a growing body of evidence from experimental studies shows that F. vesiculosus has phenotypic plasticity and tolerance against salinity change (Rothäusler et al., 2018; Rugiu et al., 2018a, b), and genetic studies show that different sibling groups of F. vesiculosus have different responses to environmental change, including OAW (Al-Janabi et al., 2016a, b). There may also be population-specific responses to different stressors, especially if populations are genetically isolated. In a study performed in the Danish Straits, certain populations of F. vesiculosus were only slightly affected by a salinity decline, while others displayed clearer responses; one population even showed severe stress symptoms and stopped growing (Kinnby et al., 2020).
It has also been shown that Fucus radicans, an endemic congener of F. vesiculosus, which is tolerant to low salinity, might be able to occupy the niche of F. vesiculosus in the northernmost Baltic if salinity declines (Rugiu et al., 2018a). If F. radicans can replace the ecological functions of its congener, its increase may potentially delay or modify the most drastic consequences of climate change on the invertebrate and fish species dependent on bladderwrack belts. Changes in species interactions involved in climate-induced environmental changes are however very difficult to project. Some studies project a decrease of grazers of Fucus spp. in the northern areas (Kotta et al., 2019), while others predict an increase (Leidenberger et al., 2015).
Similar experiments on climate change effects to those done with bladderwrack have also been made with other macroalgae and certain vascular plants. In field mesocosm experiments, OA increased the growth of the opportunistic green alga Ulva intestinalis in the Kõiguste Bay, Gulf of Riga, Estonia (Pajusalu et al., 2013, 2016). This suggests that OA could favour such fast-growing species and induce an overgrowth of Fucus sp. by annual green algae.
Salinity decline is projected to decrease the distributional ranges of the marine eelgrass Zostera marina and the red alga Furcellaria lumbricalis (Torn et al., 2020). The changing environment poses an evolutionary risk for populations that live close to the limits of their geographical ranges (Johannesson et al., 2011), including Z. marina (Billingham et al., 2003). Indeed, mesocosm studies have indicated that, while OA has little effect on the eelgrass Zostera marina (Pajusalu et al., 2015), they suffer from heatwaves in summer (Ehlers et al., 2008) and elevated temperatures in winter-spring period (Sawall et al., 2021). On the other hand, the viability of eelgrass beds also strongly depends on water clarity. A study performed for the southernmost Baltic Sea coupled biogeochemical and species distribution modelling to assess how projected wind fields, hydrodynamic conditions and nutrient abatement scenarios affect the distribution of eelgrass Z. marina in the future (2062–2066). It was concluded that nutrient reductions that fulfil the Baltic Sea Action Plan of the Helsinki Commission (HELCOM BSAP) will lead to an expansion of eelgrass coverage, despite potentially harmful effects on eelgrass distribution caused by the climate change (Bobsien et al., 2021).
Certain species may be favoured by the projected climate change. Lowering of salinity generally favours vascular plants originating from freshwater, and temperature increase favours thermophilic species, such as charophytes (Torn et al., 2020). In mesocosm studies made in Kõiguste Bay, photosynthesis of charophytes (Chara aspera, C. tomentosa and C. horrida) increased under high pCO2 treatments (Pajusalu et al., 2015), which suggests that they may be favoured by ocean acidification.
To sum up, recent studies suggest that changes in species composition of macroalgae and vascular plants are likely due to temperature, pH and salinity changes. Climate change, in conjunction with other environmental changes (especially eutrophication) may also influence carbon storage in both macroalgae and vascular plants in the Baltic Sea (Röhr et al., 2016; Takolander et al., 2017a; Jonsson et al., 2018; Salo et al., 2020; Bobsien et al., 2021). It has been projected that macroalgae will decline in hard bottoms and vascular plants increase in the more sheltered soft-bottom areas (Torn et al., 2020). Because algae and plants mostly occupy different habitats, the possible increase of vascular plants or charophytes cannot counteract the negative effects of the disappearance of macroalgae from hard-bottom areas. Consequently the invertebrates, fish and birds benefiting from habitats formed by macroalgae also will suffer from the climate change.
As with other species groups, projecting the fate of macroalgae and vascular plants is challenging. This is caused by the uncertainties in projections concerning salinity and stratification (Lehmann et al., 2022), discrepancy on which physicochemical factors determine the distribution of invertebrates, unknown adaptation capabilities of algae and plants, and uncertainties concerning future trophic interactions within macroalgae and vascular plant communities.
4.6 Benthic invertebrates
Soft-bottom benthic communities are dependent on several hydrographic and biogeochemical variables, and parameters that change with climatic variations have been shown to drive the long-term progression of zoobenthic communities (Weigel et al., 2015; Rousi et al., 2019; Ehrnsten, 2020). In the SW coast of Finland, a drastic community change took place, with amphipods being replaced by Baltic clam Limecola balthica and the non-indigenous polychaete Marenzelleria spp. This major community change was explained by an increase in near-bottom temperature and by fluctuations in salinity and oxygen (Rousi et al., 2013). In the Åland Islands (northern Baltic Sea), zoobenthos variations in 1983–2012 were associated with salinity decline, and environmentally driven shifts in the links between zoobenthos and benthic-feeding fish assemblages were recorded (Snickars et al., 2015). Long-term climate-induced shifts in zoobenthos and other trophic levels have also been described in various parts of the Baltic Sea (Törnroos et al., 2019; Forsblom et al., 2021). In all these cases, interactions between the physicochemical climate-affected parameters and secondary impacts (mainly eutrophication and/or hypoxia) have been identified.
Many marine invertebrates will directly and indirectly suffer from decreasing salinity. In experiments simulating projected changes in temperature and salinity, the survival of the isopod Idotea balthica decreased, albeit with differences between and within regions (Rugiu et al., 2018c). In addition, effects of warming on invertebrates are often non-linear, with stress effects being manifested after a certain threshold. In experiments, respiration and growth of the isopod Idotea balthica first increased until 20 ∘C and then decreased at 25 ∘C (Ito et al., 2019). Heatwaves, which have been projected to increase in frequency (Meier et al., 2019a), may therefore pose a severe threat to sublittoral invertebrates (Pansch et al., 2018). Different species show different responses to single and sequential heatwaves, however, resulting in a change in community structure. For example, the bivalve Limecola balthica suffered from repeated heatwaves, whereas the sessile amphipod Corophium sp. benefited from them. The polychaete Polydora cornuta seemed to get acclimated to heatwaves when they were repeated, showing some signs of adaptation. In general, heatwaves favoured crawling or burrowing predators and suspension feeders, while the abundance of detritivores decreased, suggesting a climate-induced change in dominant zoobenthic traits (Pansch et al., 2018).
Ocean acidification has various effects on benthic invertebrates. The size and time to settlement of pelagic larvae of the Baltic clam Limecola/Macoma balthica increased in mesocosms (in the western Gulf of Finland) with OA, suggesting a developmental delay (Jansson et al., 2016), while OA had no effects on larvae of the bay barnacle Amphibalanus improvisus originating from Kiel Fjord, southern Baltic Sea (Pansch et al., 2012). Short- (12 h) or long-term (2 week) exposures to OA did not have significant effects on the isopod Saduria entomon either (Jakubowska et al., 2013). Controversial results were obtained for the isopod Idotea balthica from three different sea areas: the North Sea, Kattegat, and the Baltic Sea. The populations from the more oceanic and saline habitats were not significantly affected by OA, while the Baltic Sea population showed 100 % mortality (Wood et al., 2014). It was suggested that the Baltic I. balthica had higher levels of oxidative stress and that the combined stress became lethal to them.
Several modelling studies have suggested that climate-induced changes in temperature, salinity and eutrophication, affecting oxygen levels and food availability for benthos, drive the development of benthic communities and their biomass in the future (Ehrnsten et al., 2019a, b). A physiological fauna model linked to a 3D coupled hydrodynamic–ecological model projected that, in areas previously burdened by hypoxia, benthic biomass will increase (until year 2100) by up to 200 % after re-oxygenating bottom waters, whereas in permanently oxygenated areas the macrofauna biomass will decrease by 35 % due to lowered food supply to the benthic ecosystem (Timmermann et al., 2012). In another modelling study, zoobenthic production decreased in the coastal zones and gradually also decreased in the more offshore areas, with increasing temperature and declining salinity and bottom oxygen regardless of the nutrient load scenarios (Weigel et al., 2015). The fate of zoobenthos also depends on human intervention, i.e. success of nutrient-reduction schemes. For instance, it has been projected that if the HELCOM BSAP is implemented, the biomass of benthic animals, and hence food for benthic-eating fish, will first increase and then decrease (Ehrnsten et al., 2020).
There are very few modelling studies focusing on invertebrates inhabiting shallower hard-bottom habitats. One study, where experimental work and species distribution modelling were combined, projected a decline of the isopod Idothea balthica in the future, mainly due to the salinity-induced decline of its host macroalgae Fucus vesiculosus (Kotta et al., 2019). Another study reached quite different conclusions. Species distribution models combined with oceanographic–biogeochemical scenarios for 2050 projected an increase in habitat suitability for Idotea balthica and I. chelipes and concluded that changes in temperature and ice cover will be more important determinants for these species than changes in salinity (Leidenberger et al., 2015).
One modelling study also investigated how Saduria entomon, a cold-water crustacean that occupies brackish and limnic parts of the Baltic Sea, will be affected by climate change and eutrophication (Gogina et al., 2020). The applied models project a net increase (and some local declines) for S. entomon and conclude that the positive effects of declining salinity will override the effects of the two nutrient load scenarios (business as usual and BSAP). The success of this species in the future warmer conditions is also facilitated by its good tolerance for temperature changes.
It is notable that hypoxia, which is a key factor affecting zoobenthos, is by no means limited to the deep basins of the Baltic Sea (Conley et al., 2011). The archipelagos of the northern Baltic Sea are especially prone to hypoxia due to their complex topography and limited water exchange (Virtanen et al., 2019). Increasing sea surface temperature will strengthen stratification and enhance mineralisation of organic matter by microbes, which may increase the release of phosphorus from sediments (Puttonen et al., 2016) and lead to a “vicious circle of eutrophication” (Vahtera et al., 2007). The sheltered archipelago areas and enclosed bays may therefore become “climate change hotspots” (Queiros et al., 2021), where zoobenthic communities are most drastically changed as well.
To sum up, zoobenthic communities are affected by all environmental parameters that are projected to change with climate change, i.e., temperature, salinity, pH and oxygen, as well as benthic–pelagic coupling. However, the effects are not unidirectional, and several processes may amplify or counteract the possible changes. The magnitude of the future salinity decline is unclear, and other factors, such as decreasing ice cover and changes in future wind conditions (of which no consensus exists), may also affect nutrient and oxygen dynamics of the Baltic Sea. Also, there may be feedback effects on sediment oxygen levels, as different benthic species have different bioirrigation activities (Norkko et al., 2012; Guy-Haim et al., 2018). Such processes, which are dependent on traits of a few species, may be of particular importance in low-diversity systems such as the northern Baltic Sea (Gladstone-Gallagher et al., 2021).
4.7 Non-indigenous invertebrates
It is often suggested that global climate change favours invasions of non-indigenous species (NIS) worldwide (Jones and Cheung, 2015). This is plausible because an increase in temperature will open new niches and induce a poleward shift of the ranges of species inhabiting tropical and temperate sea areas. In the Baltic Sea, it has been shown that non-native species typically occur in areas characterised by high temperatures, reduced salinity, high proportion of soft seabed, and decreased wave exposure, whereas most native species display an opposite pattern (Jänes et al., 2017). This suggests that the former areas are more prone to climate-induced range expansion of non-native species than the latter. This is consistent with the hypothesis of climate change hotspots, which suggests that some coastal areas may be more susceptible to effects of climate change than others (Queiros et al., 2021).
Modelled scenarios of temperature and salinity have been used to project how changes in the abiotic environment could affect NIS already present in the Baltic Sea. One modelling study suggests an increase in Ponto-Caspian cladocerans in the pelagic community and an increase in dreissenid bivalves, amphipods and mysids in the coastal benthic areas of the northern Baltic Sea until 2100 (Holopainen et al., 2016).
To sum up, the global climate change induces many environmental changes that may favour establishment of NIS in the Baltic Sea. However, attribution of the observed establishments to the climate change is difficult. It has even been claimed that there is no conclusive evidence that NIS will gain significant advantage from environmental alterations caused by climate change (Henseler et al., 2021). Stochastic processes related to maritime transport and other types of human activities are obviously important for the chances of NIS to be introduced and established into a given sea area. Long-term surveys and comparisons with areas where NIS have not been established are needed to distinguish climate-related effects from other ecosystem-level drivers (Bailey et al., 2020).
4.8 Fish
Fish populations in the Baltic Sea are influenced by various environmental and anthropogenic factors, including nutrition, predation, habitat destruction, and fisheries but also by climatic variations.
Sprat probably benefits from global climate change because increasing spring and summer temperatures have in empirical studies been observed to increase survival of sprat eggs and larvae (Voss et al., 2012) and in modelling studies to increase productivity and biomass of sprat (R. Voss et al., 2011; Mackenzie et al., 2012; Niiranen et al., 2013).
For herring the results are more variable. The growth rate of herring larvae is positively affected by temperature (Hakala et al., 2003), but weight at age and stock biomass of herring adults has in several studies been linked to the availability of food, mainly determined by the abundance of marine copepods and competition with sprat (Flinkman et al., 1998; Möllmann et al., 2003; Casini et al., 2011; Heikinheimo, 2011; Otto et al., 2014b). In modelling studies both an increase (Bartolino et al., 2014) and a short-term decrease (until 1950) (Niiranen et al., 2013) of herring populations have been projected.
Both herring and sprat populations probably benefited from the eutrophication during the 1950s to 1980s (Eero et al., 2016), which is the same period that the Baltic Sea eutrophication status changed from good to poor (Andersen et al., 2017; Murray et al., 2019). Since then, sprat biomass has varied independently of nutrient dynamics and has been more strongly affected by climatic variation and top-down control, i.e. cod predation and fisheries (Eero et al., 2016).
Based on experimental and modelling studies, future climatic variations may affect Baltic cod through their effects on water temperature, salinity, oxygen, and pH, as well as nutrients, which indirectly affect both the availability and quality of food (Limburg and Casini, 2019; Möllmann et al., 2021). The responses of cod larvae to ocean acidification (OA) have been studied experimentally, also in combination with warming (OAW). In some studies, no effects of OA or OAW on hatching, survival or development rates of cod larvae were found (Frommel et al., 2013), while in others mortality of cod larvae doubled when they were treated with high-end projections of OA (based on RCP8.5). When the projected increase of mortality was included into a stock-recruitment model, recruitment of western Baltic cod declined to only 8 % of the baseline recruitment (Stiasny et al., 2016), suggesting a dramatic effect of OA on cod populations.
A thorough review including long-term data and modelling demonstrated how predation, fishing, eutrophication and climate have sequentially affected eastern Baltic cod during the past century (Eero et al., 2011). In the early decades of the 20th century, cod reproduction was successful but seal predation and food availability kept the size of cod stock at a moderate level. From the 1940s, fishing replaced seal predation in controlling cod population, whereas the slowly increasing eutrophication had a minor positive influence on cod spawning stock biomass in 1950s to 1970s. In the late 1970s, a series of large saline inflows increased the salinity of the Baltic Sea and kept oxygen conditions in the deep basins favourable for cod. Consequently, reproduction peaked in 1978–1982 and, as fishing pressure was also temporarily low, the spawning stock biomass increased to a record-breaking level of ca. 700 000 t in 1980–1984 (Eero et al., 2011). After this peak period, cod stock started to decline due to a drastic reduction of the water volume where conditions are sufficiently saline and oxic for survival of cod eggs and larvae, the “cod reproductive volume” (RV). The decline of RV was associated with a stagnation period with low oxygen caused by a combination of anthropogenic eutrophication and climate-induced paucity of major saline inflows. Since then, the productivity of cod stocks has remained low (Eero et al., 2020), and the average maximum length of cod individuals has also been constantly declining (Orio et al., 2021). The reason for low growth may have been the low availability of both benthic and pelagic food (Neuenfeldt et al., 2020). Alternatively, a long-term exposure to low-oxygen conditions may affect body chemistry (Limburg and Casini, 2019) and decrease digestion rate and food consumption of cod (Brander, 2020). The physiological hypothesis is strengthened by the observed increase in depth distribution of cod and consequent dwelling of cod in low oxygen water (Casini et al., 2021).
Several studies project low abundances of cod towards the end of the century due to the climate- and eutrophication-induced decrease of RV (Eero et al., 2011, 2020; Gårdmark et al., 2013; Niiranen et al., 2013; Wåhlström et al., 2020). It has also been speculated that seal predation could contribute to keeping cod stocks low. However, although seal predation can cause damage to cod fisheries in coastal areas (Blomquist and Waldo, 2021), it has been concluded that the increased seal predation is a less important factor for the future size of fish stocks in the Baltic Sea than climate, eutrophication and fisheries (Mackenzie et al., 2011; Tomczak et al., 2021).
There is some disagreement on the effect of fisheries on cod stocks in the future. Earlier studies suggested that fisheries limitations may well enable stock recovery even in a “cod-hostile” environment (Cardinale and Svedäng, 2011; Heikinheimo, 2011). Certain recent modelling studies have however been less optimistic and projected that cod productivity will remain low due to the large impact of environmental drivers, especially oxygen and availability of food (Eero et al., 2020). For the western Baltic cod (inhabiting the Danish straits and the Arkona Sea) it has even been suggested that cod is now beyond a tipping point, with severe ecological, economic, and social consequences. At a critical moment, fisheries management failed to fully consider the changed environmental conditions, and climatic factors now prevent the recovery of cod stocks (Möllmann et al., 2021).
Increasing seawater temperature has also made it possible for certain warm-water Atlantic species, such as anchovy (Alheit et al., 2012), sole and turbot (Sparrevohn et al., 2013) to occur more abundantly in Kattegat and the southernmost Baltic Sea. Such northward and eastward migrations of these warm-water species may be caused by both global climate change and by variations in the Northern Hemisphere temperature anomalies (NHA), North Atlantic Oscillation (NAO), the Atlantic Multidecadal Oscillation (AMO), as well as contraction of the subpolar gyre (Alheit et al., 2012; Sparrevohn et al., 2013).
As for coastal freshwater fish, the distribution of pikeperch (Sander lucioperca) expanded towards the north along the coasts of the Bothnian Sea, apparently due to the warming of waters (Pekcan-Hekim et al., 2011). For many coastal piscivores (perch, pike, pike-perch) and cyprinids, eutrophication status of coastal waters is however a more important factor for distribution than climatic variation (Snickars et al., 2015; Bergström et al., 2016). A long-term study covering 4 decades (1970s to 2010s) made at different coastal areas of the Baltic Sea illustrated that it is hard to disentangle the effects of abiotic factors from biotic interactions affecting fish and their benthic food-sources (Törnroos et al., 2019).
To sum up, temperature, salinity, oxygen and pH have a big impact on Baltic fish recruitment and growth, and as all these variables respond to climatic variations it seems evident that fish communities in the Baltic Sea will undergo changes, with the open-sea ecosystem remaining dominated by clupeids and certain freshwater fish increasing in coastal areas (Reusch et al., 2018; Stenseth et al., 2020; Möllmann et al., 2021). Together with other environmental changes, especially eutrophication, changes in fish populations may lead to altered food web dynamics (Eero et al., 2021), necessitating ecosystem-based management of fisheries and socio-ecological adaptation (Woods et al., 2021).
The Baltic Sea ecosystem is impacted by climate-induced changes in the physical and biogeochemical environment in various ways. Climatic changes affect species and populations directly and indirectly, also impacting micro-evolution of species and having synergistic effects on other environmental drivers such as eutrophication and hypoxia (Wikner and Andersson, 2012; Niiranen et al., 2013; Ehrnsten et al., 2020; Pecuchet et al., 2020; Schmidt et al., 2020). In synergy, these impacts have already boosted the emergence of “novelty” in the system and profoundly altered pathways of energy (Ammar et al., 2021). This development will probably continue, at least if the environmental conditions of the Baltic Sea continue to change as projected by modelling studies. Below, recent findings regarding climate impacts on structure and functioning on the Baltic Sea ecosystem are summarised.
5.1 Projections of primary production and eutrophication
For the global ocean it has been projected that climate change will decrease both primary and secondary production because of intensified stratification and decreased availability of nutrients in the surface layer (Blanchard et al., 2012; IPCC, 2019). The effects of climate change on the Baltic Sea ecosystem may however be different because of the special hydrographical characteristics, peculiar communities, strong seasonal cycle, and the strong dependency of the Baltic Sea of both its watershed and the adjacent North Sea.
In the Baltic Sea, changes in ice conditions, water temperature, density stratification, and especially supply of nutrients through rivers and from the sediment, affect the nutrient dynamics and primary productivity in both coastal areas and the open sea. Different species however respond in different ways to changes in the environmental parameters, and both increases and decreases in primary production have been reported and projected along with climate-induced changes in the environment.
Climate change will most probably mean milder winters, and if soils remain thawed, more nutrients will leak from the terrestrial areas into the freshwater system. The nutrient load into the sea will probably increase, especially in the northern Baltic Sea where precipitation is probably increasing the most (Lessin et al., 2014; Huttunen et al., 2015; Christensen et al., 2022) but also in the southern Baltic Sea (M. Voss et al., 2011). It has also been projected that the total phosphorus loading (from terrestrial areas of Finland) will increase relatively more than that of nitrogen (Huttunen et al., 2015) and, together with the internal loading of phosphorus from sediments (Lessin et al., 2014; Stigebrandt et al., 2014; Stigebrandt and Anderson, 2020), phosphorus availability to primary producers may increase. If the N:P ratio of the surface layer will decline, the spring bloom will decline and more excess phosphate will be available for the summer cyanobacteria communities after the spring bloom (Lessin et al., 2014). This hypothesis especially concerns the Baltic Proper and the Gulf of Finland and perhaps the southern Bothnian Sea as well.
In the central Baltic Sea, increased spring water temperature causes, together with increased irradiation and enhanced wind-induced mixing of the surface layer, an earlier but less intense spring bloom. In summer, in contrast, an increase in temperature is coupled with increased thermal stratification, which is projected to favour production of cyanobacteria (Meier et al., 2011a; Neumann et al., 2012; Chust et al., 2014; Andersson et al., 2015). Intensified blooms of cyanobacteria are expected especially if hypoxia will prevail and internal loading will decrease the N:P ratio (Meier et al., 2011b; Funkey et al., 2014; Lessin et al., 2014). If the biomass of diazotrophic cyanobacteria will increase, nitrogen fixation could also increase, further contributing to the decrease in the N:P ratio (Lessin et al., 2014).
Several modelling studies project an increase in total phytoplankton concentration (chlorophyll, in mg m−3), until the end of the century, with the increase manifested especially in summer (Meier et al., 2012a, b; Lessin et al., 2014; Skogen et al., 2014; Ryabchenko et al., 2016). As hypoxia and associated internal loading of phosphorus will probably be enforced by global warming (Meier et al., 2019b; Tomczak et al., 2021), it has even been suggested that this “vicious circle of eutrophication” (Vahtera et al., 2007), will prevent the success of nutrient abatement measures unless internal loading of phosphorus is reduced (Gustafsson et al., 2012; Stigebrandt and Anderson, 2020).
Nutrient abatement may however counteract climate effects. For instance, in Kattegat in mid-1990s, reduction of nutrient loading led to a shift from a highly eutrophic state, characterised by small phytoplankton species and low water transparency, to an improved state, with a larger share of diatoms, decreased phytoplankton biomass and increase in water transparency (Lindegren et al., 2012). An opposing trend has taken place in the Bothnian Sea. Because of the lack of halocline and lower anthropogenic nutrient loading, the Bothnian Sea has thus far remained in a relatively good condition. However, since the year 2000 the Bothnian Sea has also shown symptoms of eutrophication (Kuosa et al., 2017), and open-sea cyanobacteria blooms have also become more common in recent years, due to a “leaking” of phosphorus-rich water from the central Baltic Sea through the Åland Sea (Rolff and Elfwing, 2015; Ahlgren et al., 2017). The connection of this process to climate change is not certain. Rather, the severe hypoxia of the central Baltic Sea has brought the anoxic layer so close to the sill separating the Baltic Proper from the Åland Sea that flow of nutrient-rich water across the Åland Sea is at times possible. Whether or not the proceeding climate change will amplify the ongoing eutrophication of the Bothnian Sea remains to be seen, but if temperature stratification increases and N:P ratio continues to decline, this will also create conditions that are favourable for cyanobacteria blooms in this relatively pristine sea area.
Several recent modelling studies conclude that nutrient abatement according to HELCOM BSAP will in the long run counteract the climate-induced increase in nutrient loading and lead to decreased eutrophication (Meier et al., 2018; Ehrnsten et al., 2019a; Murray et al., 2019; Pihlainen et al., 2020). Based on oceanographic–biogeochemical modelling, it has also been suggested that hypoxia will eventually diminish (Meier et al., 2021) and that extreme cyanobacteria blooms will no longer occur in the future, if nutrient loadings will be lowered according to BSAP despite the proceeding climate change (Meier et al., 2019a).
To sum up, the fate of the level of primary production and level of eutrophication will depend on various intertwined factors and processes and on the development of both the climate and society. Changes in primary production will impact interactions between the main trophic levels, i.e. phytoplankton, detritus, zoobenthos, detritivores, benthivores, grazers, zooplanktivores and piscivores (Kiljunen et al., 2020; Kortsch et al., 2021).
5.2 Trophic efficiency and consequences to the secondary production
Recycling and build-up of carbon within the ecosystem determines the overall productivity and biomass of different trophic levels. Several studies suggest fundamental changes in trophic dynamics and eventually in the pathways of carbon in the Baltic Sea.
A climate- and nutrient-load-driven model reconstruction of the Baltic Sea state from 1850 to 2006 suggests that the shift from spring to summer primary production is accompanied by an intensification of pelagic recycling of organic matter (Gustafsson et al., 2012). In mesocosm studies, warming accelerated (southern Baltic Sea) phytoplankton spring bloom and increased carbon-specific primary productivity (Sommer and Lewandowska, 2011; Sommer et al., 2012; Paul et al., 2016). The total phytoplankton biomass decreased because increased stratification decreased nutrient flux to the surface layer however (Lewandowska et al., 2012, 2014). Furthermore, in stratified conditions the relative importance of pathways of carbon through the microbial food web increased because copepods switched to feed more on ciliates than phytoplankton. Decrease in ciliates in turn increased the amount of heterotrophic nanoflagellates grazing on bacteria. Decreases in bacteria may reduce remineralisation and thus decrease availability of nutrients for phytoplankton (Lewandowska et al., 2014). On the other hand, decreasing of bacteria would also decrease competition for nutrients between bacteria and phytoplankton, which could counteract the negative effects of diminishing remineralisation on phytoplankton.
It has also been projected that, in addition to nutrients, the flow of dissolved organic matter (DOM) into the Baltic Sea will increase in the future climate (M. Voss et al., 2011; Strååt et al., 2018). Precipitation will increase, especially in the northern areas, and by using long-term time series from 1994 to 2006, it was shown that climate change has increased discharge of terrestrial DOM into the middle part of the Gulf of Bothnia. This provided additional substrate for bacteria, which maintained bacterial biomass production despite reduced phytoplankton production (Wikner and Andersson, 2012). This suggests that increased humic-rich river inflow may counteract climate change induced eutrophication in the coastal waters (Andersson et al., 2013).
Experimental studies have also demonstrated increased microbial activity and biomass with increasing DOM and temperature (Ducklow et al., 2010), although different bacteria taxa respond differently to the simultaneous increase of DOM and temperature (Lindh et al., 2015). Increase of DOM and bacteria may be detrimental to primary production as bacteria compete for nutrients with phytoplankton and as the brownification of water reduces light availability. Consequently, the carbon flow shifts towards microbial heterotrophy, which may induce a decrease in both phytoplankton productivity and biomass and lead to a promotion of the microbial food web and other heterotrophic organisms (Wikner and Andersson, 2012; Andersson et al., 2013). Especially if stratification increases, cycling of carbon through the microbial food web increases pelagic recycling and may also decrease vertical flux of organic matter to zoobenthos (Ehrnsten et al., 2020).
It has been suggested that climate change may also decrease fish productivity. In areas where climate change increases the supply of allochthonous DOM into the system, and where increasing stratification reduces the transport of nutrients from deeper waters, phytoplankton production may decline and the trophic pathways from bacteria and flagellates through ciliates to copepods may strengthen (Aberle et al., 2015). When the system shifts towards heterotrophy, the food web efficiency declines (Båmstedt and Wikner, 2016), and if zooplankton also becomes dominated by smaller-sized plankton (Dahlgren et al., 2011; Suikkanen et al., 2013; Jansson et al., 2020), there will be less suitable food available for planktivorous fish. If sedimentation of organic matter will also be reduced, zoobenthos production will decrease and there will be less food for benthic-eating fish. Eventually the total fish production may decrease.
Results of experimental studies have not equivocally confirmed this hypothesis. A study performed in a large biotest area artificially heated by the cooling waters of the Forsmark nuclear power plant, in the southern Bothnian Sea, found that warming of water may lead to increased species turnover and decreased compositional stability of diatom, macrophyte and invertebrate communities (Hillebrand et al., 2010). Certain mesocosm studies, simulating effects of climate change in the pelagic ecosystem, have also found that the production and biomass of both copepods and fish (three-spined sticklebacks) can remain high because the positive effects of increasing temperature and increasing availability of DOC override the negative effects of decreasing food web efficiency on copepod production (Lefébure et al., 2013).
Furthermore, many Baltic Sea copepods are omnivorous and can opportunistically switch between suspension feeding on flagellates and raptorial feeding on ciliates (Kiørboe et al., 1996). Such a flexible feeding strategy stabilises the system and can sustain copepod production even under lower phytoplankton production. This flexibility, and the fact that heterotrophic production increases with high DOC availability, suggests that fish production may be supported even when a relatively large amount of carbon flows through the microbial food web (Lefébure et al., 2013).
To sum up, a reorganisation of pathways of carbon is possible in the Baltic Sea due to the climate change. However, the system is complex due to several counteracting and interacting processes and large uncertainties in key processes, such as stratification and nutrient loads from land and the sediments (Meier et al., 2019c; Saraiva et al., 2019), and both increases and decreases of secondary producers have been demonstrated in field, experimental and modelling studies. The complexity of the system has been highlighted by a thorough review that illustrated how changes in benthic–pelagic coupling may induce ecosystem-wide consequences via increasing sedimentation of organic matter, inducing hypoxic conditions and internal loading of nutrients (Griffiths et al., 2017).
5.3 Food web interactions in the sublittoral ecosystem
If climate change induces an increase in allochthonous nutrient loads, consequences can be expected in the communities of algae and vascular plants in the shallow photic zone. The shallow-water food webs based on macroalgae and seagrasses may also be affected by the indirect effects of climate change, mediated through interactions between algae and their grazers.
The effects of late summer heatwaves on algae and invertebrates living amongst bladderwrack Fucus vesiculosus have been studied by outdoor mesocosm experiments (Werner et al., 2016). A heatwave resulted in a collapse of invertebrate grazers, such as isopods and amphipods, which in turn released grazing on filamentous algae and resulted in overgrowth of Fucus by epiphytic algae. In the autumn and winter, when the biomass of epiphytes was lower, the process was reversed: warming resulted in intensified grazing on bladderwrack. Again, a significant reduction in Fucus biomass resulted (Werner et al., 2016). As for the microalgae (diatoms), growing on Fucus in spring, temperature effects were stronger than grazing effects, suggesting a positive overall effect of climate change on microalgae (Werner and Matthiessen, 2017).
Similar results were obtained in an artificially heated biotest basin (Forsmark nuclear power plant) in the Gulf of Bothnia, where the biomass of the non-native gastropod grazer Potamopyrgus, gammarids and the snail Theodoxus were much higher than in the adjacent non-heated area. The community shift was mainly driven by direct temperature effects on invertebrates and by indirect effects of changes in vegetation cover (Salo et al., 2020). Cascading effects are also possible. In the same biotest basin, perch shifted from feeding on small fish to gammarid crustaceans, which released grazing pressure from filamentous algae (Svensson et al., 2017). If similar cascades take place in other coastal sea areas of the Baltic Sea as well, warming may promote the growth of filamentous algae and contribute to the decline of bladderwrack.
Decline of bladderwrack will affect other species due to declining availability to habitat and food (Takolander et al., 2017a; Jonsson et al., 2018; Kotta et al., 2019). Connectivity between bladderwrack populations and organisms inhabiting patches of bladderwrack may also decline (Jonsson et al., 2020; Virtanen et al., 2020). However, perhaps due to the complex biotic interactions in the sublittoral ecosystem, there are very few modelling studies that have attempted to project the fate of the algal and invertebrate communities inhabiting the shallow photic zone of the Baltic Sea. Only one study has used a combination of experimental work and modelling to study the effects of climate change on invertebrates. A decline of the isopod Idothea baltica, the main grazer of Fucus spp., was projected due to the decline of bladderwrack (Kotta et al., 2019).
To sum up, temperature and salinity changes have been projected to affect species interactions in hard and soft bottoms in the sublittoral zone. Both summer heatwaves and cold season warming can induce novel trophic interactions that produce eutrophication-like effects, e.g. overgrowth of bladderwrack by epiphytes, in the photic zone dominated by macroalgae, even without an increase in nutrient loading. However, as macroalgae are very much dependent on water clarity, the future level of eutrophication will also affect the fate of the shallow-water communities in the Baltic Sea. The complexity of the system, uncertainty of the oceanographic projections, and unknown adaptation capabilities of species, make it challenging to project the future food web interactions in the sublittoral ecosystem.
5.4 Regime shifts
In the 1980s a partly climate-induced regime shift was recorded with drastic changes in the central Baltic food web, including phytoplankton, zooplankton and pelagic planktivores and their main predator, Baltic cod (Möllmann et al., 2009; Lindegren et al., 2010a). In 1980–2000, a decline in “reproductive volume” (RV), contributed to the decline of cod population (Hinrichsen et al., 2011; Casini et al., 2016; Bartolino et al., 2017) and induced cascading effects on planktivorous fish and zooplankton (Casini et al., 2008). The different effects of temperature and salinity on sprat and cod (see above) also resulted in a spatial mismatch between these species, which further released sprat from cod predation and contributed to the increase of sprat stocks in the central Baltic Sea (Eero et al., 2012; Reusch et al., 2018). As herring is an inferior competitor for food, and food availability per individual declined, the condition of herring declined (Möllmann et al., 2003; Casini et al., 2010). Transition to a lower saline Baltic Sea, and associated decline of marine copepods (Hänninen et al., 2015), also contributed to the observed halving of (3-year-old) herring weight at age, from 50–70 g in the late 1970s to 25–30 g in the 2000s (Dippner et al., 2019). The described regime shift has also been partly questioned, as the descriptions of the shift did not cover the entire food web (Yletyinen et al., 2016).
A factor that has been less often considered when studying reasons of cod decline is the interaction with another benthic predator, flounder. Flounder may be both prey for larger cod and a competitor for the small and juvenile ones. Now that cod size has declined, cod predation on flounder has decreased, releasing competition for benthic food again. This has caused more spatial overlap between flounder populations and the remaining small sized cod, and created more intense competition between flounder and the small-sized cod, further contributing to the decline in body condition of cod (Orio et al., 2020).
Multi-species modelling studies have concluded that both fishing and climate strongly affects the size of cod stocks. If fishing is intense but climate remains unchanged, cod declines but not very dramatically, while if climate change proceeds as projected (according to the intermediate-high A2 scenario), cod goes extinct in two models out of seven, even with the present low fishing effort (Gårdmark et al., 2013). Different combinations of climate change and eutrophication scenarios may however yield very different outcomes. Medium CO2 concentrations (RCP4.5), low nutrients and sustainable fisheries resulted in high biodiversity and high numbers of cod and flounder, while high emissions (RCP8.5) and high nutrient loads resulted in diminished biodiversity and high abundance of clupeids, especially sprat (Bauer et al., 2018, 2019; Hyytiäinen et al., 2019).
The above studies have mostly considered the ecosystem of the central Baltic. In other basins, the associated processes and species interactions may be different. For example, in the Bothnian Bay, salinity was also a major driver for changes in populations of planktivorous fish, but the species involved were different. Here the decline of spawning-stock biomass of herring, observed in 1980–2013, was explained by a simultaneously increased competition with vendace, a limnic species that had increased with lowering salinity (Pekcan-Hekim et al., 2016).
In Kattegat, the western Baltic Sea, where the ecosystem is more oceanic than in the other parts of the Baltic Sea, a regime shift was detected in mid-1990s. Here the shift was explained by global climate change, cyclic climate phenomena, and human intervention. First, a reduction in anthropogenic nutrient loading led into a shift from a eutrophic ecosystem state to an ecosystem characterised by decreasing phytoplankton and zooplankton biomass, dominance by small-sized fish in the pelagial, and an increase in macroalgae and filter-feeding molluscs on hard bottoms and other benthic animals in the soft sediments (Lindegren et al., 2012). Second, the positive phases of NAO and BSI enabled an inflow of oxygenised water from the North Sea, which improved conditions for zoobenthos, including the commercially important Norway lobster. A climate-induced increase of sea surface temperatures contributed to the improved flatfish growth and survival in the shallow nursery areas (Lindegren et al., 2012). Decreasing fishing may also have contributed to the increase of gadoid and flatfish populations, but its relative importance is difficult to distinguish from other co-occurring effects.
To sum up, regime shifts are usually a result of several environmental, climatic and anthropogenic effects acting synergistically on the entire ecosystem. The climate-driven changes in temperature and salinity have been identified as key drivers for the significant rise of “novelty” in both abiotic conditions and biotic assemblages in several basins of the Baltic Sea (Ammar et al., 2021), but human contributions, i.e., anthropogenic eutrophication or its alleviation, have been a factor (Reusch et al., 2018). The recent research confirms that climate change induces multiple direct and indirect effects on species and communities and affects nutrient and carbon dynamics of the Baltic Sea ecosystem. However, despite the major structural changes, the overall food web complexity in the central Baltic Sea has remained surprisingly stable (Yletyinen et al., 2016). The relatively small changes may be explained by the fact that responses to climate change are not uniform or unidirectional but vary from species group to another, within groups and even between sibling species. Species-specific responses, many feedbacks, altered trophic pathways and the possibility of species-level adaptation combine to make projections concerning the state of the ecosystem and trophic effects challenging.
The main challenge when analysing effects of climate change on the Baltic Sea is the possible synergistic effects of climate with other environmental drivers, such as eutrophication, harmful substances and the introduction of non-indigenous species, which also may have profound impacts on ecosystems and their functioning (Reusch et al., 2018; Stenseth et al., 2020; Bonsdorff, 2021). Consequently there are numerous knowledge gaps, bottlenecks and issues of dissensus that weaken our ability to project the future biological processes, such as primary and secondary productivity, benthic–pelagic coupling and hypoxia, interactions between phytoplankton, zooplankton, and fish populations; and geographic shifts in macroalgal and invertebrate communities.
Attribution of the observed phenomena to climate change is challenging because of the collinear, intertwined and interacting processes. It is especially difficult to distinguish the effects of anthropogenic global climate change from those of quasi-cyclic phenomena, such as the NAO or BSI, or from other more stochastic variations in climate. This is partly due to the slow pace of climatic variations and time lags between physical and chemical variations and ecosystem responses. A fairly small number of studies have investigated a period long enough to cover any larger number of NAO periods. Research into the long-term dynamics of the food webs in particular is still scarce (Törnroos et al., 2019; Pecuchet et al., 2020; Kortsch et al., 2021).
Field studies have ended up with different conclusions concerning past and present changes of the environment and the biota and their causes depending on time periods and data scrutinised. For instance, certain studies note that cyanobacteria have increased (Suikkanen et al., 2013; Kuosa et al., 2017), while others do not find proof for such a phenomenon (Griffiths et al., 2020; Olofsson et al., 2020). Different periods studied, sparse sampling, varying species responses, and changes in phenology rather than total biomass, may explain some of the discrepancies between studies. The tendency of filamentous cyanobacteria to float during calm weather may also bias our view on the total biomass cyanobacteria in the sea, especially if low wind periods become more frequent.
Experimental studies are useful in pinpointing causative relationships, but their small spatial scales, short duration and simple food webs make upscaling of results to natural systems difficult. Experiments usually only last for a few days or weeks and study one or few species at a time. Reproducing natural patterns of environmental variability is also challenging. When mesocosms of hundreds of litres and natural communities are used, it may be difficult to simulate seasonal processes extending over several life cycles of the studied organisms. Even the most sophisticated multi-stressor experiments, which use levels of environmental stressors projected by modelling studies, tend to use constant stress levels.
A few mesocosm studies have exposed the communities to near-natural environmental conditions and have been able to shed light on the complex dynamics of the Baltic Sea ecosystem, e.g. the responses of the microbial food web to changes of environmental variables affected by the climate change. In studies made in the Gulf of Bothnia, bacterial, phytoplankton and zooplankton production increased with additions of inorganic carbon, and the systems remained net autotrophic. In contrast, when both nutrients and DOC was increased, only bacterial and zooplankton production increased, driving the system to net heterotrophy (Andersson et al., 2013; Båmstedt and Wikner, 2016). Increased heterotrophy led to a decreased fatty acid content and lower individual weight in the zooplankton (Dahlgren et al., 2011). With the combined treatment of elevated temperature and terrestrial nutrient loads, fish production (of three-spined sticklebacks) also increased, with terrestrial rather than autotrophic carbon being the main energy source (Lefébure et al., 2013). The complex responses indicate that to provide useful inferences about physiological and population-level responses of organisms to climate change, experimental work should use full communities, apply naturalistic exposure regimes, and investigate effects of stress at spatial and temporal scales appropriate to the species studied (Gunderson et al., 2016).
Ecosystem modelling using coupled oceanographic–biogeochemical models has advanced greatly in the past 15 years, but significant challenges remain. Projections of sea surface temperature and ice conditions can be held as relatively reliable, but there are still large uncertainties in projecting salinity, stratification, hypoxia and hence the rate of internal loading (Meier et al., 2022a). Also, natural variability is a larger source of uncertainty in future projections of hypoxia than previously understood (Meier et al., 2021). Because salinity, stratification and oxygen strongly affect many Baltic Sea organisms, it is difficult to project the fate of plankton and benthos communities with certainty. This uncertainty especially concerns marine species such as cod, bladderwrack, eelgrass, and blue mussel, which in many studies have been projected to decrease in the northern basins of the Baltic Sea. Further, uncertainties are imposed by complex biogeochemical processes in the terrestrial and freshwater ecosystems, as well as by unknown development of national economies and farming practices (Huttunen et al., 2015), especially in coastal areas strongly affected by nutrient loading.
Ecosystem models rarely consider complex biological interactions and feedback effects caused, for example, by multi-species predatory or intraguild relationships. Inclusion of such effects would require parameterizing the 3D ecosystem models with experiments and results from multi-species food web models that operate on the level populations rather than carbon flows. Also, models cannot at present consider potential adaptation capabilities of species, as little is known about them. Several recent studies have however pointed out, for example, that macroalgae (Rothäusler et al., 2018; Rugiu et al., 2018a) and zooplankton (Karlsson and Winder, 2020) have phenotypic plasticity and potential for adaptation against gradual changes in the abiotic environment.
Food web models offer useful tools for assessing the relative effects of climate, eutrophication and other human impacts, including fisheries, on the structure of the Baltic Sea ecosystem. They could potentially take into account characteristics of species and their responses to changes in the environment. The current models however mostly concern the pelagic ecosystems (e.g. cod–sprat–herring–zooplankton food chain) and there are major gaps for key trophic groups, such as macrophytes and macrozoobenthos (Korpinen et al., 2022) and the microbial food web.
The 3D ecosystem models, food web models and 2D spatial modelling would benefit from integration. Species distribution models (SDMs) can be produced at a fine spatial scale, even a few tens of metres (Virtanen et al., 2018), and in climate change studies they can be parameterised with 3D model results (Jonsson et al., 2018; Kotta et al., 2019). In the future, food web models involving relevant coastal taxa could also be used to fill in the missing links between the large-scale (3D) processes and detailed spatial patterns identified by the 2D models.
Assessing climate effects in a smaller spatial scale would be useful because shallow and sheltered bays, lagoons and estuaries may be more susceptible to climate change effects than deeper offshore areas, and may appear as “climate change hotspots”, where climate change drives the ecosystem towards a new state (Queiros et al., 2021). The existing coupled oceanographic–biogeochemical modelling studies however typically have a horizontal resolution of 1 or 2 nautical miles (ca. 2 or 4 km) and thus cannot easily be used for projecting local variations in temperature, salinity and stratification within the archipelago or inside estuaries. A bottleneck for high-resolution 3D models is the poor availability of high-resolution pan-Baltic bathymetries and forcing data (e.g. wind fields). For the SDMs, in turn, a major constraint is in many areas the poor availability of detailed species and habitat mapping data, as well as availability of high-resolution data on benthic substrates. Considering population-level effects on spatial patterns of species would also require estimation of connectivity between sea areas, a research field that is also underdeveloped in the Baltic Sea (Berglund et al., 2012; Jonsson et al., 2020; Virtanen et al., 2020). Consequently, no study has thus far considered how climate change affects microclimatic patterns in the Baltic Sea or how different species and habitats may respond to such local variations.
Due to the above challenges, there are certain discrepancies concerning our view on the effects of climate change on the structure and function of the Baltic Sea ecosystem. Some of these issues are highlighted below.
Increased primary production and phytoplankton biomass (measured in chlorophyll a) have been projected by several modelling studies (Meier et al., 2012a; Skogen et al., 2014; Ryabchenko et al., 2016). Experimental studies however show that responses of phytoplankton to climate-induced changes in temperature, salinity and pH are variable and can be modified by simultaneous changes in biogeochemical processes and zooplankton grazers (Paul et al., 2015; Sommer et al., 2015). Also, strengthening of stratification and simultaneous increase in riverine DOM loads may induce a decrease in phytoplankton production, at least in sea areas where rivers carry large DOM loads (Wikner and Andersson, 2012). Presently it is not clear which of these processes determine primary production in different sea areas or whether there are transition areas where the two processes balance each other, leading to no net change in primary production.
Several ecosystem models also predict an increase of cyanobacteria. As cyanobacteria blooms are favoured by warm, stabile and conditions and low N:P ratios (Munkes et al., 2021), increases in vigorous blooms and an increase in nitrogen fixation could be expected. However, there are large differences in model projections due to the unclear relationship between excess phosphorus and cyanobacteria growth, and the relation between bloom intensity and nitrogen fixation (Munkes et al., 2021). Cyanobacteria are also not a uniform group. Some cyanobacteria species benefit from increased temperature and acidification, whereas others suffer due to them (Eichner et al., 2014; Berner et al., 2018; Paul et al., 2018). Further uncertainty is caused by unknown biological factors, such as stoichiometric elasticity and the impact of viruses and grazers. Thus, it is still challenging to project how biomasses of cyanobacteria and nitrogen fixation will develop in the future.
Recent awareness of marine heatwaves and their potential impacts on the marine ecosystem has increased our knowledge on how climate change may impact pelagic, benthic and littoral communities in the ocean (Pansch et al., 2018; Saha et al., 2020). More studies on the responses of pelagic and benthic organisms of the Baltic Sea to heatwaves would increase our understanding of the population-level consequences of short-term variability in environmental parameters. Research on effects of climate change would also benefit from methodological diversity. For example, more extensive use of biochemical and genetic methods, such as biomarkers (Turja et al., 2014, 2015; Villnäs et al., 2019), stable isotopes (Voss et al., 2000; Gorokhova et al., 2005; Morkune et al., 2016; Lienart et al., 2021), compound-specific isotope analyses (Ek et al., 2018; Weber et al., 2021) or metabarcoding (Leray and Knowlton, 2015; Bucklin et al., 2016; Klunder et al., 2022), as well as development of remote sensing methods (Huber et al., 2021), could yield novel information on stress levels experienced by organisms and environmental niches preferred by species. Such information would allow validation of the biogeochemical models under different environmental and climate scenarios.
There is some bias in the focus organisms and habitats studied. While experiments on planktonic organisms and soft-bottom animals are relatively abundant, experiments on macroalgae, vascular plants and invertebrates inhabiting hard bottoms are less abundant, and studies focusing on the entire food web are scarce. In general, empirical and modelling studies focusing on climate effects on shallow photic habitats are less abundant than those on the pelagic and deep benthic habitats (Tedesco et al., 2016). Very few studies have investigated the shallow water ecosystems holistically, including macroalgae and microalgae, invertebrates, and fish at the same time. Those that have done so have revealed complex interactions and multiple feedbacks between species and ecosystem components (Svensson et al., 2017; Salo et al., 2020). Also, while there are ample monitoring data on pelagic and deep benthic communities, similar long-term records are very sporadic for communities associated with key habitat-forming species such as bladderwrack, eelgrass, blue mussel on hard bottoms, and vascular plants growing on soft sediments. This lack of empirical data and subsequent modelling studies hampers our understanding of the long-term responses of sublittoral communities to climate change.
Furthermore, there is a large body of literature published on sea ice algae and sea ice ecology in the Baltic Sea (Granskog et al., 2006; Tedesco et al., 2017; Thomas et al., 2017), and all of them are relevant for studying winter ecology. However, few of them have directly assessed the effects of climate change on ice ecology in the Baltic Sea. More empirical and modelling studies including quantitative projections on the effect of diminishing sea ice to biodiversity and functioning of the Baltic Sea ecosystem in winter and spring would therefore be desirable.
To sum up, there are still several significant knowledge gaps and issues of dissensus in our understanding of the effects of climate change on the Baltic Sea ecosystem. To fill these gaps, the results and conclusions from the experimental work should be better integrated into the wider empirical and modelling studies of food web dynamics, and more emphasis should be placed on studying effects of climate change on less studied environments, such as the microbial food web, sea ice communities and the sublittoral ecosystem. Such studies would provide a more comprehensive view of the responses of the pelagic and benthic systems to climate change in both the open sea and the benthic system, from bacteria to fish (Kortsch et al., 2021). Also, continuation of both spatial mapping programmes and long-term ecological studies will be crucial for validating experimental results and for developing ecosystem models, advancing our understanding of environmental and meteorological drivers of the Baltic Sea ecosystem on large spatial and temporal scales.
Climate change has an obvious potential to affect entire marine food webs, from coastal to offshore areas, from shallow to deep waters and from pelagic to benthic systems. Climate change can also induce changes in species distributions and proportions, and key nodes and linkages in the food webs may be altered or lost (Lindegren et al., 2010b; Niiranen et al., 2013; Leidenberger et al., 2015; Griffiths et al., 2017; Kotta et al., 2019; Gårdmark and Huss, 2020). As many ecosystem services are dependent on the state of the entire ecosystem (Hyytiäinen et al., 2019), a long-term decline in provision of ecosystem services to humans is possible. It is therefore indispensable to increase our understanding of the consequences of climate change on the socio-ecological system of the Baltic Sea and its surrounding marine regions (Stenseth et al., 2020).
The direct and indirect effects of climate-change-related parameters on species, communities and the ecosystem are summarised in Table 1 based on research done since 2010. While results are variable, some conclusions can be drawn from the evidence this far.
Table 1Summary of research findings and conclusions on the anticipated effects of climate change (CC) effects in the Baltic Sea for selected variables. The table only shows studies published in 2011–2021 and some of the studies referred to in the text are not included. For earlier studies, see Dippner et al. (2008) and Viitasalo et al. (2015). Observations, experimental simulations or modelled projections are categorised using the following abbreviations: T stands for temperature increase, S stands for salinity decline, TSO2 stands for temperature increase, salinity decline and/or oxygen decline, A stands for acidification, AT stands for acidification and temperature increase, AS stands for acidification increase and salinity decline, DOM stands for dissolved organic matter. EXP stands for experimental manipulations /microcosms, MES stands for experimental manipulations /mesocosms, LTS stands for long-term studies, MOD stands for modelling studies, FIE stands for field data. Empty fields indicate knowledge gaps.
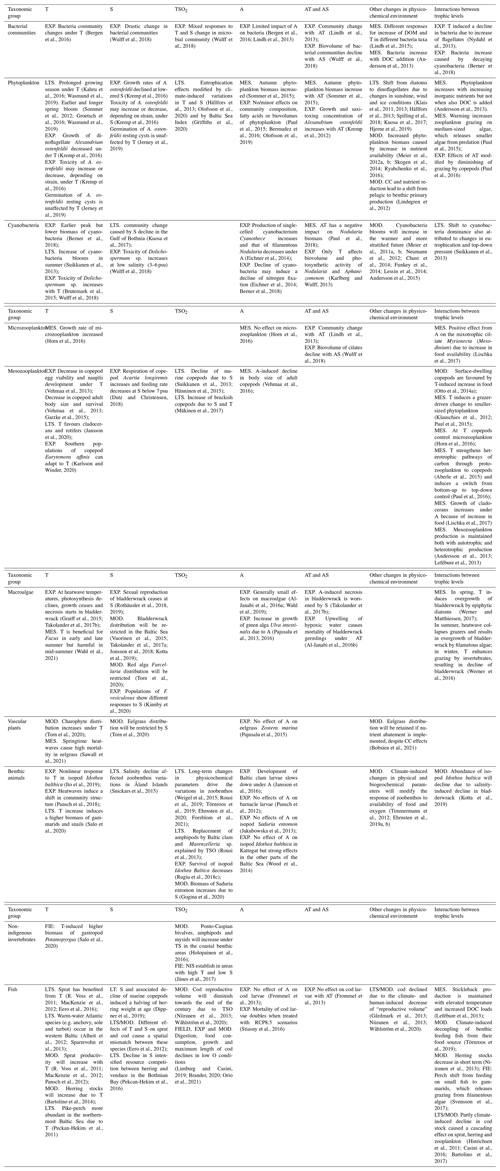
As for the eutrophication status of the Baltic Sea, it can be concluded that the ecological status of the Baltic Sea has not significantly improved despite a decrease in anthropogenic nutrient loading since the 1980s (Fleming-Lehtinen et al., 2015; Andersen et al., 2017), largely due to the pervasive internal loading (Murray et al., 2019; Stigebrandt and Anderson, 2020). Success of nutrient abatement largely determines the future state of the Baltic Sea (Hyytiäinen et al., 2019; Ehrnsten et al., 2020), but climate change may delay or even counter the improvement of the ecosystem state (Bonsdorff, 2021).
Climate-induced increases of nutrient loading and enhancing of internal loading of phosphorus have been hypothesised to promote phytoplankton and cyanobacteria production and to maintain the “vicious circle of eutrophication” (Vahtera et al., 2007), and several modelling studies indeed project an increase in both total phytoplankton biomass and cyanobacteria blooms in the future (Meier et al., 2011a; Funkey et al., 2014).
The eutrophication process may however be counteracted by various factors. Increase of DOM flowing via the rivers may decrease both primary and secondary production, at least in the Gulf of Bothnia (Wikner and Andersson, 2012; Andersson et al., 2013), and certain cyanobacteria may be negatively affected by increased temperature and ocean acidification (Paul et al., 2018). Thus, changes in structure and functioning of phytoplankton and cyanobacteria communities are probable, but the narrative that global climate change will inevitably increase phytoplankton biomass and cyanobacteria blooms and amplify the eutrophication of the Baltic Sea, may be too simplistic and needs to be refined by reconsidering the climate effects on food web processes and nutrient and carbon dynamics.
For the deep benthic communities, climate change effects are also not straightforward. If salinity declines, the majority of marine species will suffer, but according to the latest analyses undisputable evidence is lacking for a future decline in the salinity of the Baltic Sea (Lehmann et al., 2022; Meier et al., 2022b). Improvement of oxygen conditions may first promote higher zoobenthos biomasses, but eventually increasing stratification will weaken benthic–pelagic coupling and reduce food availability for benthic organisms. If nutrient abatement also proceeds favourably, biomass of zoobenthos will start to decline (Ehrnsten et al., 2020).
In the shallower photic benthic systems, nutrient increase probably enhances eutrophication, and if salinity also declines, habitat-forming marine species, such as bladderwrack, eelgrass and blue mussel, probably decline in the northern Baltic Sea (Vuorinen et al., 2015; Jonsson et al., 2018; Kotta et al., 2019). As both eutrophication and increasing temperature favour filamentous algae, continued major changes in the sublittoral communities can be expected, including negative effects of such algal aggregations (Arroyo and Bonsdorff, 2016). Of particular concern is the potential loss from rocky substrates of the habitat-forming bladderwrack and red macroalgae. Freshwater vascular plants will be favoured by freshening of the Baltic Sea, but they cannot replace the marine macroalgae on rocky sublittoral because they only grow on soft substrates. On the other hand, salinity projections are still uncertain (Lehmann et al., 2022), and even if salinity declined, Fucus vesiculosus may be able to adapt to salinity changes (Rothäusler et al., 2018).
As for fish, responses also depend on species. Salinity decline and hypoxia increase will most probably have negative consequences on cod stocks (Gårdmark et al., 2013), whereas the increasing temperature has been projected to favour sprat (Mackenzie et al., 2012) and certain coastal fish (Bergström et al., 2016). Again, as projections for salinity, stratification and oxygen levels are uncertain, the future fate of fish populations cannot be projected with certainty.
The global climate change induces many environmental changes that may favour establishment of NIS in the Baltic Sea. Opportunistic and thermophilic species occupying soft sediments are the most probable winners. It is notable that it is extremely difficult to eradicate a marine NIS after it has found a suitable niche in the Baltic Sea. As the effects of NIS on both the ecosystem and the society are usually negative, their spreading should be prevented already before they enter the Baltic Sea, by effectively eradicating NIS from ballast waters of ships and other possible vectors.
Climate change is obviously not the only factor determining the fate of the Baltic Sea in the future. Several modelling studies have concluded that nutrient reductions will be a stronger driver for ecosystem functions in the Baltic Sea than climate change (Friedland et al., 2012; Niiranen et al., 2013; Ehrnsten et al., 2019a; Pihlainen et al., 2020; Meier et al., 2021). In moderate nutrient loading scenarios climate change will also play a role, but under full implementation of BSAP, the environmental state of the Baltic Sea is projected to become significantly improved and hypoxia reduced by the end of the century (Meier et al., 2018, 2021; Saraiva et al., 2018, 2019). Despite the many uncertainties concerning the effects of climate and eutrophication on the state of the Baltic Sea (Munkes et al., 2021), it can be concluded that continued abatement of anthropogenic nutrient loading, combined with sustainable fisheries, seem to be the most reliable, albeit slow, measures to solve the grand challenges of the Baltic Sea (Meier et al., 2018; Murray et al., 2019).
Several studies have focused on studying the effects of climate change on the future state of the Baltic Sea, and the ecosystem modelling studies already provide valuable results that are directly usable in decision-making concerning mitigation of eutrophication under climate change. In contrast, studies concerning effects of climate change on biodiversity of the Baltic Sea are lagging behind and are hampered by model uncertainties (e.g. for salinity) and by the current inability of models to consider the complex interactions between species and multiple feedbacks between trophic levels. Long-term and modelling studies focusing on shallow photic environments, which harbour the highest biodiversity in the Baltic Sea, are especially sparse. This is a major drawback in a situation where all major environmental policies, including the UN Convention on Biological Diversity and EU Biodiversity Strategy for 2030, urge for halting ongoing biodiversity loss. To designate effective measures to safeguard biodiversity, including a climate-smart expansion of the protected area network, a better understanding of the effects of climate change on the sublittoral ecosystem is urgently needed.
Knowledge of the mechanisms and processes governing the Baltic Sea ecosystem under climate change have recently accumulated and already provide information that can be used to design adaptation tools and mitigation measures for the Baltic Sea (Reusch et al., 2018). It is necessary to continue studying the Baltic Sea as a socio-ecological system, responding to both environmental and societal changes (Bauer et al., 2018, 2019; Hyytiäinen et al., 2019), and to continue the dialogue with human society, in order to attune to the future changes ultimately driven by the ocean itself (Stenseth et al., 2020).
This review paper refers exclusively to published research articles and their data. We refer the reader to the cited literature for access to data.
MV designed the review article, wrote main parts of the paper, produced the table and edited the manuscript versions for review. EB contributed with texts and comments.
The contact author has declared that neither they nor their co-author has any competing interests.
Publisher's note: Copernicus Publications remains neutral with regard to jurisdictional claims in published maps and institutional affiliations.
This article is part of the special issue “The Baltic Earth Assessment Reports (BEAR)”. It is not associated with a conference.
This work has been done under the auspices of the Finnish Environment Institute, Marine Research Centre (Markku Viitasalo) and is a part of the Åbo Akademi University Strategic Profile “The Sea” (Erik Bonsdorff). We thank the Baltic Earth secretariat for inviting us to write this review and three anonymous reviewers for constructive comments significantly improving earlier versions of the manuscript.
This research has been supported by the projects SmartSea (Academy of Finland, Strategic Research Council, grant nos. 292985 and 314225) and FutureMARES (EU Horizon 2020, grant no. 869300) for Markku Viitasalo and by the Åbo Akademi Foundation for Erik Bonsdorff.
This paper was edited by Markus Meier and reviewed by three anonymous referees.
Aberle, N., Malzahn, A. M., Lewandowska, A. M., and Sommer, U.: Some like it hot: the protozooplankton-copepod link in a warming ocean, Mar. Ecol. Prog. Ser., 519, 103–113, https://doi.org/10.3354/meps11081, 2015.
Ahlgren, J., Grimvall, A., Omstedt, A., Rolff, C., and Wikner, J.: Temperature, DOC level and basin interactions explain the declining oxygen concentrations in the Bothnian Sea, J. Marine Syst., 170, 22–30, https://doi.org/10.1016/j.jmarsys.2016.12.010, 2017.
Alheit, J., Pohlmann, T., Casini, M., Greve, W., Hinrichs, R., Mathis, M., O'Driscoll, K., Vorberg, R., and Wagner, C.: Climate variability drives anchovies and sardines into the North and Baltic Seas, Prog. Oceanogr., 96, 128–139, https://doi.org/10.1016/j.pocean.2011.11.015, 2012.
Al-Janabi, B., Kruse, I., Graiff, A., Karsten, U., and Wahl, M.: Genotypic variation influences tolerance to warming and acidification of early life-stage Fucus vesiculosus L. (Phaeophyceae) in a seasonally fluctuating environment, Mar. Biol., 163, 14, https://doi.org/10.1007/s00227-015-2804-8, 2016a.
Al-Janabi, B., Kruse, I., Graiff, A., Winde, V., Lenz, M., and Wahl, M.: Buffering and amplifying interactions among OAW (Ocean Acidification and Warming) and nutrient enrichment on early life-stage Fucus vesiculosus L. (Phaeophyceae) and their carry over effects to hypoxia impact, PLoS ONE, 11, e0152948, https://doi.org/10.1371/journal.pone.0152948, 2016b.
Ammar, Y., Niiranen, S., Otto, S. A., Möllmann, C., Finsinger, W., and Blenckner, T.: The rise of novelty in marine ecosystems: The Baltic Sea case, Glob. Change Biol., 27, 1485–1499, https://doi.org/10.1111/gcb.15503, 2021.
Andersen, J. H., Carstensen, J., Conley, D. J., Dromph, K., Fleming-Lehtinen, V., Gustafsson, B. G., Josefson, A. B., Norkko, A., Villnäs, A., and Murray, C.: Long-term temporal and spatial trends in eutrophication status of the Baltic Sea, Biol. Rev., 92, 135–149, https://doi.org/10.1111/brv.12221, 2017.
Andersson, A., Jurgensone, I., Rowe, O. F., Simonelli, P., Bignert, A., Lundberg, E., and Karlsson, J.: Can Humic Water Discharge Counteract Eutrophication in Coastal Waters?, PLoS ONE, 8, 13, https://doi.org/10.1371/journal.pone.0061293, 2013.
Andersson, A., Meier, H. E. M., Ripszam, M., Rowe, O., Wikner, J., Haglund, P., Eilola, K., Legrand, C., Figueroa, D., Paczkowska, J., Lindehoff, E., Tysklind, M., and Elmgren, R.: Projected future climate change and Baltic Sea ecosystem management, Ambio, 44, S345–S356, https://doi.org/10.1007/s13280-015-0654-8, 2015.
Arroyo, N. L. and Bonsdorff, E.: The role of drifting algae for marine biodiversity, in: Marine macrophytes as foundation species, edited by: Olafsson, E., CRC Press, Taylor and Francis Group, 100–123, ISBN 978-1-4987-2324-4, 2016.
Bailey, S. A., Brown, L., Campbell, M. L., Canning-Clode, J., Carlton, J. T., Castro, N., Chainho, P., Chan, F. T., Creed, J. C., Curd, A., Darling, J., Fofonoff, P., Galil, B. S., Hewitt, C. L., Inglis, G. J., Keith, I., Mandrak, N. E., Marchini, A., McKenzie, C. H., Occhipinti-Ambrogi, A., Ojaveer, H., Pires-Teixeira, L. M., Robinson, T. B., Ruiz, G. M., Seaward, K., Schwindt, E., Son, M. O., Therriault, T. W., and Zhan, A. B.: Trends in the detection of aquatic non-indigenous species across global marine, estuarine and freshwater ecosystems: A 50 year perspective, Divers. Distrib., 26, 1780–1797, https://doi.org/10.1111/ddi.13167, 2020.
Båmstedt, U. and Wikner, J.: Mixing depth and allochthonous dissolved organic carbon: controlling factors of coastal trophic balance, Mar. Ecol. Prog. Ser., 561, 17–29, https://doi.org/10.3354/meps11907, 2016.
Barange, M., Merino, G., Blanchard, J. L., Scholtens, J., Harle, J., Allison, E. H., Allen, J. I., Holt, J., and Jennings, S.: Impacts of climate change on marine ecosystem production in societies dependent on fisheries, Nat. Clim. Change, 4, 211–216, https://doi.org/10.1038/nclimate2119, 2014.
Bartolino, V., Margonski, P., Lindegren, M., Linderholm, H. W., Cardinale, M., Rayner, D., Wennhage, H., and Casini, M.: Forecasting fish stock dynamics under climate change: Baltic herring (Clupea harengus) as a case study, Fish. Oceanogr., 23, 258–269, https://doi.org/10.1111/fog.12060, 2014.
Bartolino, V., Tian, H. D., Bergström, U., Jounela, P., Aro, E., Dieterich, C., Meier, H. E. M., Cardinale, M., Bland, B., and Casini, M.: Spatio-temporal dynamics of a fish predator: Density-dependent and hydrographic effects on Baltic Sea cod population, PLoS ONE, 12, e0172004, https://doi.org/10.1371/journal.pone.0172004, 2017.
Bauer, B., Meier, H. E. M., Casini, M., Hoff, A., Margonski, P., Orio, A., Saraiva, S., Steenbeek, J., and Tomczak, M. T.: Reducing eutrophication increases spatial extent of communities supporting commercial fisheries: a model case study, ICES J. Mar. Sci., 75, 1306–1317, https://doi.org/10.1093/icesjms/fsy003, 2018.
Bauer, B., Gustafsson, B. G., Hyytiäinen, K., Meier, H. E. M., Müller-Karulis, B., Saraiva, S., and Tomczak, M. T.: Food web and fisheries in the future Baltic Sea, Ambio, 48, 1337–1349, https://doi.org/10.1007/s13280-019-01229-3, 2019.
Belkin, I. M.: Rapid warming of Large Marine Ecosystems, Prog. Oceanogr., 81, 207–213, https://doi.org/10.1016/j.pocean.2009.04.011, 2009.
Bergen, B., Endres, S., Engel, A., Zark, M., Dittmar, T., Sommer, U., and Jürgens, K.: Acidification and warming affect prominent bacteria in two seasonal phytoplankton bloom mesocosms, Environ. Microbiol., 18, 4579–4595, https://doi.org/10.1111/1462-2920.13549, 2016.
Berglund, M., Jacobi, M. N., and Jonsson, P. R.: Optimal selection of marine protected areas based on connectivity and habitat quality, Ecol. Model., 240, 105–112, https://doi.org/10.1016/j.ecolmodel.2012.04.011, 2012.
Bergström, L., Heikinheimo, O., Svirgsden, R., Kruze, E., Lozys, L., Lappalainen, A., Saks, L., Minde, A., Dainys, J., Jakubaviciute, E., Adjers, K., and Olsson, J.: Long term changes in the status of coastal fish in the Baltic Sea, Estuar. Coast. Shelf Sci., 169, 74–84, https://doi.org/10.1016/j.ecss.2015.12.013, 2016.
Bermúdez, R., Winder, M., Stuhr, A., Almén, A.-K., Engström-Öst, J., and Riebesell, U.: Effect of ocean acidification on the structure and fatty acid composition of a natural plankton community in the Baltic Sea, Biogeosciences, 13, 6625–6635, https://doi.org/10.5194/bg-13-6625-2016, 2016.
Berner, C., Bertos-Fortis, M., Pinhassi, J., and Legrand, C.: Response of microbial communities to changing climate conditions during summer cyanobacterial blooms in the Baltic Sea, Front. Microbiol., 9, 1562, https://doi.org/10.3389/fmicb.2018.01562, 2018.
Billingham, M. R., Reusch, T. B. H., Alberto, F., and Serrao, E. A.: Is asexual reproduction more important at geographical limits? A genetic study of the seagrass Zostera marina in the Ria Formosa, Portugal, Mar. Ecol. Prog. Ser., 265, 77–83, https://doi.org/10.3354/meps265077, 2003.
Blanchard, J. L., Jennings, S., Holmes, R., Harle, J., Merino, G., Allen, J. I., Holt, J., Dulvy, N. K., and Barange, M.: Potential consequences of climate change for primary production and fish production in large marine ecosystems, Philos. T. Roy. Soc. B, 367, 2979–2989, https://doi.org/10.1098/rstb.2012.0231, 2012.
Blenckner, T., Möllmann, C., Stewart Lowndes, J., Griffiths, J., Campbell, E., De Cervo, A., Belgrano, A., Boström, C., Fleming, V., Frazier, M., Neuenfeldt, A., Niiranen, S., Nilsson, A., Ojaveer, H., Olsson, J., Palmlöv, C. S., Quaas, M., Rickels, W., Sobek, A., Viitasalo, M., Wikström, S. A., and Halpern, B. S.: The Baltic Health Index (BHI): assessing the socialecological status of the Baltic Sea, People and Nature, 3, 359–375, https://doi.org/10.1002/pan3.10178, 2021.
Blindow, I., Dahlke, S., Dewart, A., Flugge, S., Hendreschke, M., Kerkow, A., and Meyer, J.: Long-term and interannual changes of submerged macrophytes and their associated diaspore reservoir in a shallow southern Baltic Sea bay: influence of eutrophication and climate, Hydrobiologia, 778, 121–136, https://doi.org/10.1007/s10750-016-2655-4, 2016.
Blomquist, J. and Waldo, S.: Seal interactions and exits from fisheries: insights from the Baltic Sea cod fishery, ICES J. Mar. Sci., 78, 2958–2966, https://doi.org/10.1093/icesjms/fsab173, 2021.
Bobsien, I. C., Hukriede, W., Schlamkow, C., Friedland, R., Dreier, N., Schubert, P. R., Karez, R., and Reusch, T. B. H.: Modeling eelgrass spatial response to nutrient abatement measures in a changing climate, Ambio, 50, 400–412, https://doi.org/10.1007/s13280-020-01364-2, 2021.
Bonsdorff, E.: Eutrophication: Early warning signals, ecosystem-level and societal responses, and ways forward, Ambio, 50, 753–758, https://doi.org/10.1007/s13280-020-01432-7, 2021.
Brander, K.: Reduced growth in Baltic Sea cod may be due to mild hypoxia, ICES J. Mar. Sci., 77, 2003–2005, https://doi.org/10.1093/icesjms/fsaa041, 2020.
Brierley, A. S. and Kingsford, M. J.: Impacts of climate change on marine organisms and ecosystems, Curr. Biol., 19, R602–R614, https://doi.org/10.1016/j.cub.2009.05.046, 2009.
Brutemark, A., Engström-Öst, J., Vehmaa, A., and Gorokhova, E.: Growth, toxicity and oxidative stress of a cultured cyanobacterium (Dolichospermum sp.) under different CO2/pH and temperature conditions, Phycol. Res., 63, 56–63, https://doi.org/10.1111/pre.12075, 2015.
Bucklin, A., Lindeque, P. K., Rodriguez-Ezpeleta, N., Albaina, A., and Lehtiniemi, M.: Metabarcoding of marine zooplankton: prospects, progress and pitfalls, J. Plankton Res., 38, 393–400, https://doi.org/10.1093/plankt/fbw023, 2016.
Burrows, M. T., Bates, A. E., Costello, M. J., Edwards, M., Edgar, G. J., Fox, C. J., Halpern, B. S., Hiddink, J. G., Pinsky, M. L., Batt, R. D., Molinos, J. G., Payne, B. L., Schoeman, D. S., Stuart-Smith, R. D., and Poloczanska, E. S.: Ocean community warming responses explained by thermal affinities and temperature gradients, Nat. Clim. Change, 9, 959–963, https://doi.org/10.1038/s41558-019-0631-5, 2019.
Cardinale, M. and Svedäng, H.: The beauty of simplicity in science: Baltic cod stock improves rapidly in a “cod hostile” ecosystem state, Mar. Ecol. Prog. Ser., 425, 297–301, https://doi.org/10.3354/meps09098, 2011.
Casini, M., Lövgren, J., Hjelm, J., Cardinale, M., Molinero, J. C., and Kornilovs, G.: Multi-level trophic cascades in a heavily exploited open marine ecosystem, P. Roy. Soc. B-Biol. Sci., 275, 1793–1801, https://doi.org/10.1098/rspb.2007.1752, 2008.
Casini, M., Bartolino, V., Molinero, J. C., and Kornilovs, G.: Linking fisheries, trophic interactions and climate: threshold dynamics drive herring Clupea harengus growth in the central Baltic Sea, Mar. Ecol. Prog. Ser., 413, 241–252, https://doi.org/10.3354/meps08592, 2010.
Casini, M., Kornilovs, G., Cardinale, M., Mollmann, C., Grygiel, W., Jonsson, P., Raid, T., Flinkman, J., and Feldman, V.: Spatial and temporal density dependence regulates the condition of central Baltic Sea clupeids: compelling evidence using an extensive international acoustic survey, Popul. Ecol., 53, 511–523, https://doi.org/10.1007/s10144-011-0269-2, 2011.
Casini, M., Kall, F., Hansson, M., Plikshs, M., Baranova, T., Karlsson, O., Lundström, K., Neuenfeldt, S., Gårdmark, A., and Hjelm, J.: Hypoxic areas, density-dependence and food limitation drive the body condition of a heavily exploited marine fish predator, Roy. Soc. Open Sci., 3, 15, https://doi.org/10.1098/rsos.160416, 2016.
Casini, M., Hansson, M., Orio, A., and Limburg, K.: Changes in population depth distribution and oxygen stratification are involved in the current low condition of the eastern Baltic Sea cod (Gadus morhua), Biogeosciences, 18, 1321–1331, https://doi.org/10.5194/bg-18-1321-2021, 2021.
Christensen, O. B., Kjellström, E., Dieterich, C., Gröger, M., and Meier, H. E. M.: Atmospheric regional climate projections for the Baltic Sea region until 2100, Earth Syst. Dynam., 13, 133–157, https://doi.org/10.5194/esd-13-133-2022, 2022.
Chust, G., Allen, J. I., Bopp, L., Schrum, C., Holt, J., Tsiaras, K., Zavatarelli, M., Chifflet, M., Cannaby, H., Dadou, I., Daewel, U., Wakelin, S. L., Machu, E., Pushpadas, D., Butenschon, M., Artioli, Y., Petihakis, G., Smith, C., Garcon, V., Goubanova, K., Le Vu, B., Fach, B. A., Salihoglu, B., Clementi, E., and Irigoien, X.: Biomass changes and trophic amplification of plankton in a warmer ocean, Glob. Change Biol., 20, 2124–2139, https://doi.org/10.1111/gcb.12562, 2014.
Conley, D. J., Carstensen, J., Aigars, J., Axe, P., Bonsdorff, E., Eremina, T., Haahti, B. M., Humborg, C., Jonsson, P., Kotta, J., Lannegren, C., Larsson, U., Maximov, A., Medina, M. R., Lysiak-Pastuszak, E., Remeikaite-Nikiene, N., Walve, J., Wilhelms, S., and Zillen, L.: Hypoxia is increasing in the coastal zone of the Baltic Sea, Environ. Sci. Technol., 45, 6777–6783, https://doi.org/10.1021/es201212r, 2011.
Dahlgren, K., Wiklund, A. K. E., and Andersson, A.: The influence of autotrophy, heterotrophy and temperature on pelagic food web efficiency in a brackish water system, Aquat. Ecol., 45, 307–323, https://doi.org/10.1007/s10452-011-9355-y, 2011.
Dippner, J. W., Vuorinen, I., Daunys, D., Flinkman, J., Halkka, A., Köster, F. W., Lehikoinen, E., MacKenzie, B. R., Möllmann, C., Möhlenberg, F., Olenin, S., Schiedek, D., Skov, H., and Wasmund, N.: Climate-related marine ecosystem change, in: Assessment of Climate Change for the Baltic Sea Basin, edited by: BACC_Author_Group, Springer, Berlin, 309–377, eISBN 978-3-540-72786-6, 2008.
Dippner, J. W., Frundt, B., and Hammer, C.: Lake or sea? The unknown future of central Baltic Sea herring, Front. Evol. Ecol., 7, 143, https://doi.org/10.3389/fevo.2019.00143, 2019.
Doney, S. C., Ruckelshaus, M., Duffy, J. E., Barry, J. P., Chan, F., English, C. A., Galindo, H. M., Grebmeier, J. M., Hollowed, A. B., Knowlton, N., Polovina, J., Rabalais, N. N., Sydeman, W. J., and Talley, L. D.: Climate change impacts on marine ecosystems, in: Annual Review of Marine Science, Vol. 4, edited by: Carlson, C. A. and Giovannoni, S. J., Annu. Rev. Mar. Sci., Annual Reviews, Palo Alto, 11–37, https://doi.org/10.1146/annurev-marine-041911-111611, 2012.
Ducklow, H. W., Morán, X. A. G., and Murray, A. E.: Bacteria in the greenhouse: marine microbes and climate change, in: Environmental Microbiology, edited by: Mitchell, R. and Gu, J.-D., Wiley-Blackwell, Hoboken, New Jersey, 1–31, ISBN 978-0-470-17790-7, 2010.
Dutheil, C., Meier, H., Gröger, M., and Börgel, F.: Understanding past and future sea surface temperature trends in the Baltic Sea, Clim. Dynam., 1–19, 2021.
Dutz, J. and Christensen, A. M.: Broad plasticity in the salinity tolerance of a marine copepod species, Acartia longiremis, in the Baltic Sea, J. Plankton Res., 40, 342–355, https://doi.org/10.1093/plankt/fby013, 2018.
Eero, M., MacKenzie, B. R., Köster, F. W., and Gislason, H.: Multi-decadal responses of a cod (Gadus morhua) population to human-induced trophic changes, fishing, and climate, Ecol. Appl., 21, 214–226, https://doi.org/10.1890/09-1879.1, 2011.
Eero, M., Vinther, M., Haslob, H., Huwer, B., Casini, M., Storr-Paulsen, M., and Köster, F. W.: Spatial management of marine resources can enhance the recovery of predators and avoid local depletion of forage fish, Conserv. Lett., 5, 486–492, https://doi.org/10.1111/j.1755-263X.2012.00266.x, 2012.
Eero, M., Andersson, H. C., Almroth-Rosell, E., and MacKenzie, B. R.: Has eutrophication promoted forage fish production in the Baltic Sea?, Ambio, 45, 649–660, https://doi.org/10.1007/s13280-016-0788-3, 2016.
Eero, M., Cardinale, M., and Storr-Paulsen, M.: Emerging challenges for resource management under ecosystem change: Example of cod in the Baltic Sea, Ocean Coast. Manag., 198, 4, https://doi.org/10.1016/j.ocecoaman.2020.105314, 2020.
Eero, M., Dierking, J., Humborg, C., Undeman, E., MacKenzie, B. R., Ojaveer, H., Salo, T., and Köster, F. W.: Use of food web knowledge in environmental conservation and management of living resources in the Baltic Sea, ICES J. Mar. Sci., 78, 2645–2663, https://doi.org/10.1093/icesjms/fsab145, 2021.
Eglite, E., Wodarg, D., Dutz, J., Wasmund, N., Nausch, G., Liskow, I., Schulz-Bull, D., and Loick-Wilde, N.: Strategies of amino acid supply in mesozooplankton during cyanobacteria blooms: a stable nitrogen isotope approach, Ecosphere, 9, 20, https://doi.org/10.1002/ecs2.2135, 2018.
Ehlers, A., Worm, B., and Reusch, T. B. H.: Importance of genetic diversity in eelgrass Zostera marina for its resilience to global warming, Mar. Ecol. Prog. Ser., 355, 1–7, https://doi.org/10.3354/meps07369, 2008.
Ehrnsten, E.: Quantifying biomass and carbon processing of benthic fauna in a coastal sea – past, present and future, Dissertationes Schola Doctoralis Scientiae Circumiectalis, Alimentariae, Biologicae, 1–64, http://urn.fi/URN:ISBN:978-951-51-5629-7 (last access: 30 March 2022), 2020.
Ehrnsten, E., Bauer, B., and Gustafsson, B. G.: Combined effects of environmental drivers on marine trophic groups – a systematic model comparison, Front. Mar. Sci., 6, 492, 2019a.
Ehrnsten, E., Norkko, A., Timmermann, K., and Gustafsson, B. G.: Benthic-pelagic coupling in coastal seas – Modelling macrofaunal biomass and carbon processing in response to organic matter supply, J. Marine Syst., 196, 36–47, 2019b.
Ehrnsten, E., Norkko, A., Müller-Karulis, B., Gustafsson, E., and Gustafsson, B. G.: The meagre future of benthic fauna in a coastal sea – Benthic responses to recovery from eutrophication in a changing climate, Glob. Change Biol., 26, 2235–2250, https://doi.org/10.1111/gcb.15014, 2020.
Eichner, M., Rost, B., and Kranz, S. A.: Diversity of ocean acidification effects on marine N2 fixers, J. Exp. Mar. Biol. Ecol., 457, 199–207, https://doi.org/10.1016/j.jembe.2014.04.015, 2014.
Ek, C., Holmstrand, H., Mustajärvi, L., Garbaras, A., Bariseviciute, R., Sapolaite, J., Sobek, A., Gorokhova, E., and Karlson, A. M. L.: Using compound-specific and bulk stable isotope analysis for trophic positioning of bivalves in contaminated Baltic Sea sediments, Environ. Sci. Technol., 52, 4861–4868, https://doi.org/10.1021/acs.est.7b05782, 2018.
Engström-Öst, J., Barrett, N., Brutemark, A., Vehmaa, A., Dwyer, A., Almen, A. K., and De Stasio, B. T.: Feeding, survival, and reproduction of two populations of Eurytemora (Copepoda) exposed to local toxic cyanobacteria, J. Great Lakes Res., 43, 1091–1100, https://doi.org/10.1016/j.jglr.2017.09.009, 2017.
Eveleens Maarse, F., Salovius-Laurén, S., and Snickars, M.: Long-term changes in the phytobenthos of the southern Åland Islands, northern Baltic Sea, Nord. J. Bot., 38, 2020.
Fleming-Lehtinen, V., Andersen, J. H., Carstensen, J., Lysiak-Pastuszak, E., Murray, C., Pyhälä, M., and Laamanen, M.: Recent developments in assessment methodology reveal that the Baltic Sea eutrophication problem is expanding, Ecol. Indic., 48, 380–388, https://doi.org/10.1016/j.ecolind.2014.08.022, 2015.
Flinkman, J., Aro, E., Vuorinen, I., and Viitasalo, M.: Changes in northern Baltic zooplankton and herring nutrition from 1980s to 1990s: top-down and bottom-up processes at work, Mar. Ecol. Prog. Ser., 165, 127–136, https://doi.org/10.3354/meps165127, 1998.
Forsblom, L., Linden, A., Engström-Öst, J., Lehtiniemi, M., and Bonsdorff, E.: Identifying biotic drivers of population dynamics in a benthic-pelagic community, Ecol. Evol., 11, 4035–4045, https://doi.org/10.1002/ece3.7298, 2021.
Friedland, R., Neumann, T., and Schernewski, G.: Climate change and the Baltic Sea action plan: Model simulations on the future of the western Baltic Sea, J. Marine Syst., 105, 175–186, https://doi.org/10.1016/j.jmarsys.2012.08.002, 2012.
Frommel, A. Y., Schubert, A., Piatkowski, U., and Clemmesen, C.: Egg and early larval stages of Baltic cod, Gadus morhua, are robust to high levels of ocean acidification, Mar. Biol., 160, 1825–1834, https://doi.org/10.1007/s00227-011-1876-3, 2013.
Funkey, C. P., Conley, D. J., Reuss, N. S., Humborg, C., Jilbert, T., and Slomp, C. P.: Hypoxia sustains Cyanobacteria blooms in the Baltic Sea, Environ. Sci. Technol., 48, 2598–2602, https://doi.org/10.1021/es404395a, 2014.
Gårdmark, A. and Huss, M.: Individual variation and interactions explain food web responses to global warming, Philos. T. Roy. Soc. B, 375, 11, https://doi.org/10.1098/rstb.2019.0449, 2020.
Gårdmark, A., Lindegren, M., Neuenfeldt, S., Blenckner, T., Heikinheimo, O., Müller-Karulis, B., Niiranen, S., Tomczak, M. T., Aro, E., Wikström, A., and Möllmann, C.: Biological ensemble modeling to evaluate potential futures of living marine resources, Ecol. Appl., 23, 742–754, https://doi.org/10.1890/12-0267.1, 2013.
Garzke, J., Ismar, S. M. H., and Sommer, U.: Climate change affects low trophic level marine consumers: warming decreases copepod size and abundance, Oecologia, 177, 849–860, https://doi.org/10.1007/s00442-014-3130-4, 2015.
Gladstone-Gallagher, R. V., Hewitt, J. E., Thrush, S. F., Brustolin, M. C., Villnas, A., Valanko, S., and Norkko, A.: Identifying “vital attributes” for assessing disturbance-recovery potential of seafloor communities, Ecol. Evol., 11, 6091–6103, https://doi.org/10.1002/ece3.7420, 2021.
Gogina, M., Zettler, M. L., Wahlstrom, I., Andersson, H., Radtke, H., Kuznetsov, I., and MacKenzie, B. R.: A combination of species distribution and ocean-biogeochemical models suggests that climate change overrides eutrophication as the driver of future distributions of a key benthic crustacean in the estuarine ecosystem of the Baltic Sea, ICES J. Mar. Sci., 77, 2089–2105, https://doi.org/10.1093/icesjms/fsaa107, 2020.
Gorokhova, E., Hansson, S., Höglander, H., and Andersen, C. M.: Stable isotopes show food web changes after invasion by the predatory cladoceran Cercopagis pengoi in a Baltic Sea bay, Oecologia, 143, 251–259, https://doi.org/10.1007/s00442-004-1791-0, 2005.
Graiff, A., Liesner, D., Karsten, U., and Bartsch, I.: Temperature tolerance of western Baltic Sea Fucus vesiculosus – growth, photosynthesis and survival, J. Exp. Mar. Biol. Ecol., 471, 8–16, https://doi.org/10.1016/j.jembe.2015.05.009, 2015.
Granskog, M., Kaartokallio, H., Kuosa, H., Thomas, D. N., and Vainio, J.: Sea ice in the Baltic Sea – A review, Estuar. Coast. Shelf S., 70, 145–160, https://doi.org/10.1016/j.ecss.2006.06.001, 2006.
Griffiths, J. R., Kadin, M., Nascimento, F. J. A., Tamelander, T., Törnroos, A., Bonaglia, S., Bonsdorff, E., Bruchert, V., Gårdmark, A., Järnström, M., Kotta, J., Lindegren, M., Nordström, M. C., Norkko, A., Olsson, J., Weigel, B., Zydelis, R., Blenckner, T., Niiranen, S., and Winder, M.: The importance of benthic-pelagic coupling for marine ecosystem functioning in a changing world, Glob. Change Biol., 23, 2179–2196, https://doi.org/10.1111/gcb.13642, 2017.
Griffiths, J. R., Lehtinen, S., Suikkanen, S., and Winder, M.: Limited evidence for common interannual trends in Baltic Sea summer phytoplankton biomass, PLoS ONE, 15, e0231690, https://doi.org/10.1371/journal.pone.0231690, 2020.
Groetsch, P. M. M., Simis, S. G. H., Eleveld, M. A., and Peters, S. W. M.: Spring blooms in the Baltic Sea have weakened but lengthened from 2000 to 2014, Biogeosciences, 13, 4959–4973, https://doi.org/10.5194/bg-13-4959-2016, 2016.
Gubelit, Y. I.: Climatic impact on community of filamentous macroalgae in the Neva estuary (eastern Baltic Sea), Mar. Pollut. Bull., 91, 166–172, https://doi.org/10.1016/j.marpolbul.2014.12.009, 2015.
Gunderson, A. R., Armstrong, E. J., and Stillman, J. H.: Multiple Stressors in a changing world: The need for an improved perspective on physiological responses to the dynamic marine environment, in: Annual Review of Marine Science, Vol. 8, edited by: Carlson, C. A. and Giovannoni, S. J., Annual Review of Marine Science, Annual Reviews, Palo Alto, 357–378, https://doi.org/10.1146/annurev-marine-122414-033953, 2016.
Gustafsson, B. G., Schenk, F., Blenckner, T., Eilola, K., Meier, H. E. M., Müller-Karulis, B., Neumann, T., Ruoho-Airola, T., Savchuk, O. P., and Zorita, E.: Reconstructing the development of Baltic Sea eutrophication 1850–2006, Ambio, 41, 534–548, https://doi.org/10.1007/s13280-012-0318-x, 2012.
Guy-Haim, T., Lyons, D. A., Kotta, J., Ojaveer, H., Queiros, A. M., Chatzinikolaou, E., Arvanitidis, C., Como, S., Magni, P., Blight, A. J., Orav-Kotta, H., Somerfield, P. J., Crowe, T. P., and Rilov, G.: Diverse effects of invasive ecosystem engineers on marine biodiversity and ecosystem functions: A global review and meta-analysis, Glob. Change Biol., 24, 906–924, https://doi.org/10.1111/gcb.14007, 2018.
Hakala, T., Viitasalo, M., Rita, H., Aro, E., Flinkman, J., and Vuorinen, I.: Temporal and spatial variation in the growth rates of Baltic herring (Clupea harengus membras L.) larvae during summer, Mar. Biol., 142, 25–33, https://doi.org/10.1007/s00227-002-0933-3, 2003.
Hällfors, H., Backer, H., Leppänen, J. M., Hällfors, S., Hällfors, G., and Kuosa, H.: The northern Baltic Sea phytoplankton communities in 1903–1911 and 1993–2005: a comparison of historical and modern species data, Hydrobiologia, 707, 109–133, https://doi.org/10.1007/s10750-012-1414-4, 2013.
Hänninen, J., Vuorinen, I., Rajasilta, M., and Reid, P. C.: Response of the Baltic and North Seas to river runoff from the Baltic watershed – Physical and biological changes, Prog. Oceanogr., 138, 91–104, https://doi.org/10.1016/j.pocean.2015.09.001, 2015.
Heikinheimo, O.: Interactions between cod, herring and sprat in the changing environment of the Baltic Sea: A dynamic model analysis, Ecol. Model., 222, 1731–1742, https://doi.org/10.1016/j.ecolmodel.2011.03.005, 2011.
Henseler, C., Oesterwind, D., Kotterba, P., Nordström, M. C., Snickars, M., Törnroos, A., and Bonsdorff, E.: Impact of round goby on native invertebrate communities – An experimental field study, J. Exp. Mar. Biol. Ecol., 541, 11, https://doi.org/10.1016/j.jembe.2021.151571, 2021.
Henson, S. A., Beaulieu, C., Ilyina, T., John, J. G., Long, M., Seferian, R., Tjiputra, J., and Sarmiento, J. L.: Rapid emergence of climate change in environmental drivers of marine ecosystems, Nat. Commun., 8, 9, https://doi.org/10.1038/ncomms14682, 2017.
Hillebrand, H., Soininen, J., and Snoeijs, P.: Warming leads to higher species turnover in a coastal ecosystem, Glob. Change Biol., 16, 1181–1193, https://doi.org/10.1111/j.1365-2486.2009.02045.x, 2010.
Hine, R. (Ed.): A Dictionary of Biology, 8th edn., Oxford University Press, Oxford, ISBN 9780198821489, 2019.
Hinrichsen, H. H., Huwer, B., Makarchouk, A., Petereit, C., Schaber, M., and Voss, R.: Climate-driven long-term trends in Baltic Sea oxygen concentrations and the potential consequences for eastern Baltic cod (Gadus morhua), ICES J. Mar. Sci., 68, 2019–2028, https://doi.org/10.1093/icesjms/fsr145, 2011.
Hjerne, O., Hajdu, S., Larsson, U., Downing, A. S., and Winder, M.: Climate driven changes in timing, composition and magnitude of the Baltic Sea phytoplankton spring bloom, Front. Mar. Sci., 6, 15, https://doi.org/10.3389/fmars.2019.00482, 2019.
Hoegh-Guldberg, O. and Bruno, J. F.: The impact of climate change on the world's marine ecosystems, Science, 328, 1523–1528, https://doi.org/10.1126/science.1189930, 2010.
Hogfors, H., Motwani, N. H., Hajdu, S., El-Shehawy, R., Holmborn, T., Vehmaa, A., Engström-Öst, J., Brutemark, A., and Gorokhova, E.: Bloom-Forming Cyanobacteria support copepod reproduction and development in the Baltic Sea, PLoS ONE, 9, e112692, https://doi.org/10.1371/journal.pone.0112692, 2014.
Holopainen, R., Lehtiniemi, M., Meier, H. M., Albertsson, J., Gorokhova, E., Kotta, J., and Viitasalo, M.: Impacts of changing climate on the non-indigenous invertebrates in the northern Baltic Sea by end of the twenty-first century, Biol. Invasions, 18, 3015–3032, 2016.
Horn, H. G., Boersma, M., Garzke, J., Loder, M. G. J., Sommer, U., and Aberle, N.: Effects of high CO2 and warming on a Baltic Sea microzooplankton community, ICES J. Mar. Sci., 73, 772–782, https://doi.org/10.1093/icesjms/fsv198, 2016.
Huber, S., Hansen, L. B., Nielsen, L. T., Rasmussen, M. L., Solvsteen, J., Berglund, J., von Friesen, C. P., Danbolt, M., Envall, M., Infantes, E., and Moksnes, P.: Novel approach to large-scale monitoring of submerged aquatic vegetation: A nationwide example from Sweden, Integr. Environ. Assess. Manag., 2021, 1–12, https://doi.org/10.1002/ieam.4493, 2021.
Huttunen, I., Lehtonen, H., Huttunen, M., Piirainen, V., Korppoo, M., Veijalainen, N., Viitasalo, M., and Vehvilainen, B.: Effects of climate change and agricultural adaptation on nutrient loading from Finnish catchments to the Baltic Sea, Sci. Total Environ., 529, 168–181, https://doi.org/10.1016/j.scitotenv.2015.05.055, 2015.
Hyytiäinen, K., Bauer, B., Bly Joyce, K., Ehrnsten, E., Eilola, K., Gustafsson, B. G., Meier, H. M., Norkko, A., Saraiva, S., and Tomczak, M.: Provision of aquatic ecosystem services as a consequence of societal changes: The case of the Baltic Sea, Popul. Ecol., 63, 61–74, 2019.
IPCC: Summary for Policymakers, in: IPCC Special Report on the Ocean and Cryosphere in a Changing Climate, edited by: Pörtner, H.-O., Roberts, D. C., Masson-Delmotte, V., Zhai, P., Tignor, M., Poloczanska, E., Mintenbeck, K., Alegría, A., Nicolai, M., Okem, A., Petzold, J., Rama, B., and Weyer, N. M., Cambridge University Press, Cambridge, UK and New York, NY, USA, 3–35, https://doi.org/10.1017/9781009157964.001, 2019.
Ito, M., Scotti, M., Franz, M., Barboza, F. R., Buchholz, B., Zimmer, M., Guy-Haim, T., and Wahl, M.: Effects of temperature on carbon circulation in macroalgal food webs are mediated by herbivores, Mar. Biol., 166, 1–11, https://doi.org/10.1007/s00227-019-3596-z, 2019.
Jakubowska, M., Jerzak, M., Normant, M., Burska, D., and Drzazgowski, J.: Effect of carbon dioxide -induced water acidification on the physiological processes of the Baltic isopod Saduria entomon, J. Shellfish Res., 32, 825–834, https://doi.org/10.2983/035.032.0326, 2013.
Jänes, H., Herkül, K., and Kotta, J.: Environmental niche separation between native and non-native benthic invertebrate species: Case study of the northern Baltic Sea, Mar. Environ. Res., 131, 123–133, https://doi.org/10.1016/j.marenvres.2017.08.001, 2017.
Jansson, A., Lischka, S., Boxhammer, T., Schulz, K. G., and Norkko, J.: Survival and settling of larval Macoma balthica in a large-scale mesocosm experiment at different fCO2 levels, Biogeosciences, 13, 3377–3385, https://doi.org/10.5194/bg-13-3377-2016, 2016.
Jansson, A., Klais-Peets, R., Griniene, E., Rubene, G., Semenova, A., Lewandowska, A., and Engström-Öst, J.: Functional shifts in estuarine zooplankton in response to climate variability, Ecol. Evol., 10, 11591–11606, https://doi.org/10.1002/ece3.6793, 2020.
Jerney, J., Suikkanen, S., Lindehoff, E., and Kremp, A.: Future temperature and salinity do not exert selection pressure on cyst germination of a toxic phytoplankton species, Ecol. Evol., 9, 4443–4451, https://doi.org/10.1002/ece3.5009, 2019.
Johannesson, K., Smolarz, K., Grahn, M., and Andre, C.: The future of Baltic Sea populations: Local extinction or evolutionary rescue?, Ambio, 40, 179–190, https://doi.org/10.1007/s13280-010-0129-x, 2011.
Jones, M. C. and Cheung, W. W.: Multi-model ensemble projections of climate change effects on global marine biodiversity, ICES J. Mar. Sci., 72, 741–752, 2015.
Jonsson, P. R., Kotta, J., Andersson, H. C., Herkül, K., Virtanen, E., Nyström Sandman, A., and Johannesson, K.: High climate velocity and population fragmentation may constrain climate-driven range shift of the key habitat former Fucus vesiculosus, Divers. Distrib., 24, 892–905, https://doi.org/10.1111/ddi.12733, 2018.
Jonsson, P. R., Moksnes, P. O., Corell, H., Bonsdorff, E., and Nilsson Jacobi, M.: Ecological coherence of Marine Protected Areas: New tools applied to the Baltic Sea network, Aquat. Conserv., 30, 743–760, https://doi.org/10.1002/aqc.3286, 2020.
Kahru, M., Elmgren, R., and Savchuk, O. P.: Changing seasonality of the Baltic Sea, Biogeosciences, 13, 1009–1018, https://doi.org/10.5194/bg-13-1009-2016, 2016.
Karjalainen, M., Kozlowsky-Suzuki, B., Lehtiniemi, M., Engström-Öst, J., Kankaanpää, H., and Viitasalo, M.: Nodularin accumulation during cyanobacterial blooms and experimental depuration in zooplankton, Mar. Biol., 148, 683–691, https://doi.org/10.1007/s00227-005-0126-y, 2006.
Karjalainen, M., Engström-Öst, J., Korpinen, S., Peltonen, H., Pääkkönen, J.-P., Rönkkönen, S., Suikkanen, S., and Viitasalo, M.: Ecosystem consequences of cyanobacteria in the northern Baltic Sea, Ambio, 36, 195–202, https://doi.org/10.1579/0044-7447(2007)36[195:ecocit]2.0.co;2, 2007.
Karlberg, M. and Wulff, A.: Impact of temperature and species interaction on filamentous cyanobacteria may be more important than salinity and increased pCO2 levels, Mar. Biol., 160, 2063–2072, https://doi.org/10.1007/s00227-012-2078-3, 2013.
Karlsson, K. and Winder, M.: Adaptation potential of the copepod Eurytemora affinis to a future warmer Baltic Sea, Ecol. Evol., 10, 5135–5151, https://doi.org/10.1002/ece3.6267, 2020.
Kiljunen, M., Peltonen, H., Lehtiniemi, M., Uusitalo, L., Sinisalo, T., Norkko, J., Kunnasranta, M., Torniainen, J., Rissanen, A. J., and Karjalainen, J.: Benthic-pelagic coupling and trophic relationships in northern Baltic Sea food webs, Limnol. Oceanogr., 65, 1706–1722, https://doi.org/10.1002/lno.11413, 2020.
Kinnby, A., Jonsson, P. R., Ortega-Martinez, O., Topel, M., Pavia, H., Pereyra, R. T., and Johannesson, K.: Combining an ecological experiment and a genome scan show idiosyncratic responses to salinity stress in local populations of a seaweed, Frontiers in Marine Science, 7, 12, https://doi.org/10.3389/fmars.2020.00470, 2020.
Kiørboe, T., Saiz, E., and Viitasalo, M.: Prey switching behaviour in the planktonic copepod Acartia tonsa, Mar. Ecol. Prog. Ser., 143, 65–75, https://doi.org/10.3354/meps143065, 1996.
Klais, R., Tamminen, T., Kremp, A., Spilling, K., and Olli, K.: Decadal-scale changes of dinoflagellates and diatoms in the anomalous Baltic Sea spring bloom, PLoS ONE, 6, e21567, https://doi.org/10.1371/journal.pone.0021567, 2011.
Klais, R., Tamminen, T., Kremp, A., Spilling, K., An, B. W., Hajdu, S., and Olli, K.: Spring phytoplankton communities shaped by interannual weather variability and dispersal limitation: Mechanisms of climate change effects on key coastal primary producers, Limnol. Oceanogr., 58, 753–762, https://doi.org/10.4319/lo.2013.58.2.0753, 2013.
Klauschies, T., Bauer, B., Aberle-Malzahn, N., Sommer, U., and Gaedke, U.: Climate change effects on phytoplankton depend on cell size and food web structure, Mar. Biol., 159, 2455–2478, https://doi.org/10.1007/s00227-012-1904-y, 2012.
Klunder, L., van Bleijswijk, J. D. L., Schaars, L. K., van der Veer, H. W., Luttikhuizen, P. C., and Bijleveld, A. I.: Quantification of marine benthic communities with metabarcoding, Mol. Ecol. Resour., 22, 1043–1054, https://doi.org/10.1111/1755-0998.13536, 2022.
Kniebusch, M., Meier, H. M., Neumann, T., and Börgel, F.: Temperature variability of the Baltic Sea since 1850 and attribution to atmospheric forcing variables, J. Geophys. Res.-Oceans, 124, 4168–4187, 2019.
Korpinen, S., Honkanen, T., Vesakoski, O., Hemmi, A., Koivikko, R., Loponen, J., and Jormalainen, V.: Macroalgal communities face the challenge of changing biotic interactions: Review with focus on the Baltic Sea, Ambio, 36, 203–211, https://doi.org/10.1579/0044-7447(2007)36[203:Mcftco]2.0.Co;2, 2007.
Korpinen, S., Uusitalo, L., Nordström, M. C., Dierking, J., Tomczak, M. T., Haldin, J., Opitz, S., Bonsdorff, E., and Neuenfeldt, S.: Food web assessments in the Baltic Sea: Models bridging the gap between indicators and policy needs, Ambio, https://doi.org/10.1007/s13280-021-01692-x, in press, 2022.
Kortsch, S., Frelat, R., Pecuchet, L., Olivier, P., Putnis, I., Bonsdorff, E., Ojaveer, H., Jurgensone, I., Strake, S., Rubene, G., Kruze, E., and Nordström, M. C.: Disentangling temporal food web dynamics facilitates understanding of ecosystem functioning, J. Anim. Ecol., 90, 1205–1216, https://doi.org/10.1111/1365-2656.13447, 2021.
Kotta, J., Vanhatalo, J., Jänes, H., Orav-Kotta, H., Rugiu, L., Jormalainen, V., Bobsien, I., Viitasalo, M., Virtanen, E., and Nyström Sandman, A.: Integrating experimental and distribution data to predict future species patterns, Sci. Rep., 9, 1821, 2019.
Kremp, A., Godhe, A., Egardt, J., Dupont, S., Suikkanen, S., Casabianca, S., and Penna, A.: Intraspecific variability in the response of bloom-forming marine microalgae to changed climate conditions, Ecol. Evol., 2, 1195–1207, https://doi.org/10.1002/ece3.245, 2012.
Kremp, A., Oja, J., LeTortorec, A. H., Hakanen, P., Tahvanainen, P., Tuimala, J., and Suikkanen, S.: Diverse seed banks favour adaptation of microalgal populations to future climate conditions, Environ. Microbiol., 18, 679–691, https://doi.org/10.1111/1462-2920.13070, 2016.
Kuosa, H., Fleming-Lehtinen, V., Lehtinen, S., Lehtiniemi, M., Nygård, H., Raateoja, M., Raitaniemi, J., Tuimala, J., Uusitalo, L., and Suikkanen, S.: A retrospective view of the development of the Gulf of Bothnia ecosystem, J. Marine Syst., 167, 78–92, https://doi.org/10.1016/j.jmarsys.2016.11.020, 2017.
Lappalainen, J., Virtanen, E. A., Kallio, K., Junttila, S., and Viitasalo, M.: Substrate limitation of a habitat-forming genus Fucus under different water clarity scenarios in the northern Baltic Sea, Estuar. Coast. Shelf S., 218, 31–38, https://doi.org/10.1016/j.ecss.2018.11.010, 2019.
Lefébure, R., Degerman, R., Andersson, A., Larsson, S., Eriksson, L. O., Båmstedt, U., and Byström, P.: Impacts of elevated terrestrial nutrient loads and temperature on pelagic food-web efficiency and fish production, Glob. Change Biol., 19, 1358–1372, https://doi.org/10.1111/gcb.12134, 2013.
Lehmann, A., Myrberg, K., Post, P., Chubarenko, I., Dailidiene, I., Hinrichsen, H.-H., Hüssy, K., Liblik, T., Meier, H. E. M., Lips, U., and Bukanova, T.: Salinity dynamics of the Baltic Sea, Earth Syst. Dynam., 13, 373–392, https://doi.org/10.5194/esd-13-373-2022, 2022.
Leidenberger, S., De Giovanni, R., Kulawik, R., Williams, A. R., and Bourlat, S. J.: Mapping present and future potential distribution patterns for a meso-grazer guild in the Baltic Sea, J. Biogeogr., 42, 241–254, https://doi.org/10.1111/jbi.12395, 2015.
Leray, M. and Knowlton, N.: DNA barcoding and metabarcoding of standardized samples reveal patterns of marine benthic diversity, P. Natl. Acad. Sci. USA, 112, 2076–2081, https://doi.org/10.1073/pnas.1424997112, 2015.
Lessin, G., Raudsepp, U., Maljutenko, I., Laanemets, J., Passenko, J., and Jaanus, A.: Model study on present and future eutrophication and nitrogen fixation in the Gulf of Finland, Baltic Sea, J. Marine Syst., 129, 76–85, https://doi.org/10.1016/j.jmarsys.2013.08.006, 2014.
Lewandowska, A. M., Breithaupt, P., Hillebrand, H., Hoppe, H. G., Jürgens, K., and Sommer, U.: Responses of primary productivity to increased temperature and phytoplankton diversity, J. Sea Res., 72, 87–93, https://doi.org/10.1016/j.seares.2011.10.003, 2012.
Lewandowska, A. M., Boyce, D. G., Hofmann, M., Matthiessen, B., Sommer, U., and Worm, B.: Effects of sea surface warming on marine plankton, Ecol. Lett., 17, 614–623, https://doi.org/10.1111/ele.12265, 2014.
Liblik, T. and Lips, U.: Stratification Has Strengthened in the Baltic Sea – An Analysis of 35 Years of Observational Data, Front. Earth Sci., 7, 15, https://doi.org/10.3389/feart.2019.00174, 2019.
Lienart, C., Garbaras, A., Qvarfordt, S., Walve, J., and Karlson, A. M. L.: Spatio-temporal variation in stable isotope and elemental composition of key-species reflect environmental changes in the Baltic Sea, Biogeochemistry, 157, 149–170, https://doi.org/10.1007/s10533-021-00865-w, 2021.
Limburg, K. E. and Casini, M.: Otolith chemistry indicates recent worsened Baltic cod condition is linked to hypoxia exposure, Biol. Lett., 15, 20190352, https://doi.org/10.1098/rsbl.2019.0352, 2019.
Lindegren, M., Diekmann, R., and Möllmann, C.: Regime shifts, resilience and recovery of a cod stock, Mar. Ecol. Prog. Ser., 402, 239–253, https://doi.org/10.3354/meps08454, 2010a.
Lindegren, M., Möllmann, C., Nielsen, A., Brander, K., MacKenzie, B. R., and Stenseth, N. C.: Ecological forecasting under climate change: the case of Baltic cod, P. Roy. Soc. B-Biol. Sci., 277, 2121–2130, https://doi.org/10.1098/rspb.2010.0353, 2010b.
Lindegren, M., Blenckner, T., and Stenseth, N. C.: Nutrient reduction and climate change cause a potential shift from pelagic to benthic pathways in a eutrophic marine ecosystem, Glob. Change Biol., 18, 3491–3503, https://doi.org/10.1111/j.1365-2486.2012.02799.x, 2012.
Lindh, M. V., Riemann, L., Baltar, F., Romero-Oliva, C., Salomon, P. S., Graneli, E., and Pinhassi, J.: Consequences of increased temperature and acidification on bacterioplankton community composition during a mesocosm spring bloom in the Baltic Sea, Env. Microbiol. Rep., 5, 252–262, https://doi.org/10.1111/1758-2229.12009, 2013.
Lindh, M. V., Lefébure, R., Degerman, R., Lundin, D., Andersson, A., and Pinhassi, J.: Consequences of increased terrestrial dissolved organic matter and temperature on bacterioplankton community composition during a Baltic Sea mesocosm experiment, Ambio, 44, S402–S412, https://doi.org/10.1007/s13280-015-0659-3, 2015.
Lischka, S., Bach, L. T., Schulz, K.-G., and Riebesell, U.: Ciliate and mesozooplankton community response to increasing CO2 levels in the Baltic Sea: insights from a large-scale mesocosm experiment, Biogeosciences, 14, 447–466, https://doi.org/10.5194/bg-14-447-2017, 2017.
Loick-Wilde, N., Fernandez-Urruzola, I., Eglite, E., Liskow, I., Nausch, M., Schulz-Bull, D., Wodarg, D., Wasmund, N., and Mohrholz, V.: Stratification, nitrogen fixation, and cyanobacterial bloom stage regulate the planktonic food web structure, Glob. Change Biol., 25, 794–810, https://doi.org/10.1111/gcb.14546, 2019.
MacKenzie, B. R., Eero, M., and Ojaveer, H.: Could Seals Prevent Cod Recovery in the Baltic Sea?, PLoS ONE, 6, e18998, https://doi.org/10.1371/journal.pone.0018998, 2011.
MacKenzie, B. R., Meier, H. E. M., Lindegren, M., Neuenfeldt, S., Eero, M., Blenckner, T., Tomczak, M. T., and Niiranen, S.: Impact of climate change on fish population dynamics in the Baltic Sea: a dynamical downscaling investigation, Ambio, 41, 626–636, https://doi.org/10.1007/s13280-012-0325-y, 2012.
Mäkinen, K., Vuorinen, I., and Hänninen, J.: Climate-induced hydrography change favours small-bodied zooplankton in a coastal ecosystem, Hydrobiologia, 792, 83–96, https://doi.org/10.1007/s10750-016-3046-6, 2017.
Matthäus, W. and Schinke, H.: Mean atmospheric circulation patterns associated with major Baltic inflows, Deutsche Hydrogr. Zeitschr., 46, 321–339, 1994.
Meier, H. E. M., Eilola, K., and Almroth, E.: Climate-related changes in marine ecosystems simulated with a 3-dimensional coupled physical-biogeochemical model of the Baltic Sea, Clim. Res., 48, 31–55, https://doi.org/10.3354/cr00968, 2011a.
Meier, H. E. M., Andersson, H. C., Eilola, K., Gustafsson, B. G., Kuznetsov, I., Müller-Karulis, B., Neumann, T., and Savchuk, O. P.: Hypoxia in future climates: A model ensemble study for the Baltic Sea, Geophys. Res. Lett., 38, 1–6, https://doi.org/10.1029/2011gl049929, 2011b.
Meier, H. E. M., Hordoir, R., Andersson, H. C., Dieterich, C., Eilola, K., Gustafsson, B. G., Höglund, A., and Schimanke, S.: Modeling the combined impact of changing climate and changing nutrient loads on the Baltic Sea environment in an ensemble of transient simulations for 1961–2099, Clim. Dynam., 39, 2421–2441, https://doi.org/10.1007/s00382-012-1339-7, 2012a.
Meier, H. E. M., Müller-Karulis, B., Andersson, H. C., Dieterich, C., Eilola, K., Gustafsson, B. G., Höglund, A., Hordoir, R., Kuznetsov, I., Neumann, T., Ranjbar, Z., Savchuk, O. P., and Schimanke, S.: Impact of climate change on ecological quality indicators and biogeochemical fluxes in the Baltic Sea: a multi-model ensemble study, Ambio, 41, 558–573, https://doi.org/10.1007/s13280-012-0320-3, 2012b.
Meier, H. E. M., Edman, M. K., Eilola, K. J., Placke, M., Neumann, T., Andersson, H. C., Brunnabend, S.-E., Dieterich, C., Frauen, C., Friedland, R., Gröger, M., Gustafsson, B. G., Gustafsson, E., Isaev, A., Kniebusch, M., Kuznetsov, I., Müller-Karulis, B., Omstedt, A., Ryabchenko, V., Saraiva, S., and Savchuk, O. P.: Assessment of eutrophication Abatement acenarios for the Baltic Sea by multi-model ensemble simulations, Front. Mar. Sci., 5, 440, https://doi.org/10.3389/fmars.2018.00440, 2018.
Meier, H. E. M., Dieterich, C., Eilola, K., Gröger, M., Höglund, A., Radtke, H., Saraiva, S., and Wåhlström, I.: Future projections of record-breaking sea surface temperature and cyanobacteria bloom events in the Baltic Sea, Ambio, 48, 1362–1376, https://doi.org/10.1007/s13280-019-01235-5, 2019a.
Meier, H. E. M., Eilola, K., Almroth-Rosell, E., Schimanke, S., Kniebusch, M., Hoglund, A., Pemberton, P., Liu, Y., Vali, G., and Saraiva, S.: Disentangling the impact of nutrient load and climate changes on Baltic Sea hypoxia and eutrophication since 1850, Clim. Dynam., 53, 1145–1166, https://doi.org/10.1007/s00382-018-4296-y, 2019b.
Meier, H. E. M., Edman, M., Eilola, K., Placke, M., Neumann, T., Andersson, H. C., Brunnabend, S. E., Dieterich, C., Frauen, C., Friedland, R., Gröger, M., Gustafsson, B. G., Gustafsson, E., Isaev, A., Kniebusch, M., Kuznetsov, I., Müller-Karulis, B., Naumann, M., Omstedt, A., Ryabchenko, V., Saraiva, S., and Savchuk, O. P.: Assessment of uncertainties in scenario simulations of biogeochemical cycles in the Baltic Sea, Front. Mar. Sci., 6, 46, https://doi.org/10.3389/fmars.2019.00046, 2019c.
Meier, H. E. M., Dieterich, C., and Groger, M.: Natural variability is a large source of uncertainty in future projections of hypoxia in the Baltic Sea, Commun. Earth Environ., 2, 13, https://doi.org/10.1038/s43247-021-00115-9, 2021.
Meier, H. E. M., Dieterich, C., Gröger, M., Dutheil, C., Börgel, F., Safonova, K., Christensen, O. B., and Kjellström, E.: Oceanographic regional climate projections for the Baltic Sea until 2100, Earth Syst. Dynam., 13, 159–199, https://doi.org/10.5194/esd-13-159-2022, 2022a.
Meier, H. E. M., Kniebusch, M., Dieterich, C., Gröger, M., Zorita, E., Elmgren, R., Myrberg, K., Ahola, M. P., Bartosova, A., Bonsdorff, E., Börgel, F., Capell, R., Carlén, I., Carlund, T., Carstensen, J., Christensen, O. B., Dierschke, V., Frauen, C., Frederiksen, M., Gaget, E., Galatius, A., Haapala, J. J., Halkka, A., Hugelius, G., Hünicke, B., Jaagus, J., Jüssi, M., Käyhkö, J., Kirchner, N., Kjellström, E., Kulinski, K., Lehmann, A., Lindström, G., May, W., Miller, P. A., Mohrholz, V., Müller-Karulis, B., Pavón-Jordán, D., Quante, M., Reckermann, M., Rutgersson, A., Savchuk, O. P., Stendel, M., Tuomi, L., Viitasalo, M., Weisse, R., and Zhang, W.: Climate change in the Baltic Sea region: a summary, Earth Syst. Dynam., 13, 457–593, https://doi.org/10.5194/esd-13-457-2022, 2022b.
Möller, K. O., Schmidt, J. O., St. John, M., Temming, A., Diekmann, R., Peters, J., Floeter, J., Sell, A. F., Herrmann, J. P., and Möllmann, C.: Effects of climate-induced habitat changes on a key zooplankton species, J. Plankton Res., 37, 530–541, https://doi.org/10.1093/plankt/fbv033, 2015.
Möllmann, C., Kornilovs, G., Fetter, M., Köster, F. W., and Hinrichsen, H. H.: The marine copepod, Pseudocalanus elongatus, as a mediator between climate variability and fisheries in the Central Baltic Sea, Fish. Oceanogr., 12, 360–368, https://doi.org/10.1046/j.1365-2419.2003.00257.x, 2003.
Möllmann, C., Diekmann, R., Müller-Karulis, B., Kornilovs, G., Plikshs, M., and Axe, P.: Reorganization of a large marine ecosystem due to atmospheric and anthropogenic pressure: a discontinuous regime shift in the Central Baltic Sea, Glob. Change Biol., 15, 1377–1393, https://doi.org/10.1111/j.1365-2486.2008.01814.x, 2009.
Möllmann, C., Cormon, X., Funk, S., Otto, S. A., Schmidt, J. O., Schwermer, H., Sguotti, C., Voss, R., and Quaas, M.: Tipping point realized in cod fishery, Sci. Rep., 11, 12, https://doi.org/10.1038/s41598-021-93843-z, 2021.
Morkune, R., Lesutiene, J., Bariseviciute, R., Morkunas, J., and Gasiunaite, Z. R.: Food sources of wintering piscivorous waterbirds in coastal waters: A triple stable isotope approach for the southeastern Baltic Sea, Estuar. Coast. Shelf S., 171, 41–50, https://doi.org/10.1016/j.ecss.2016.01.032, 2016.
Moustaka-Gouni, M., Kormas, K. A., Scotti, M., Vardaka, E., and Sommer, U.: Warming and acidification effects on planktonic heterotrophic pico- and nanoflagellates in a mesocosm experiment, Protist, 167, 389–410, https://doi.org/10.1016/j.protis.2016.06.004, 2016.
Munkes, B., Löptien, U., and Dietze, H.: Cyanobacteria blooms in the Baltic Sea: a review of models and facts, Biogeosciences, 18, 2347–2378, https://doi.org/10.5194/bg-18-2347-2021, 2021.
Murray, C. J., Müller-Karulis, B., Carstensen, J., Conley, D. J., Gustafsson, B. G., and Andersen, J. H.: Past, present and future eutrophication status of the Baltic Sea, Front. Mar. Sci., 6, 12, https://doi.org/10.3389/fmars.2019.00002, 2019.
Neuenfeldt, S., Bartolino, V., Orio, A., Andersen, K. H., Andersen, N. G., Niiranen, S., Bergstrom, U., Ustups, D., Kulatska, N., and Casini, M.: Feeding and growth of Atlantic cod (Gadus morhua L.) in the eastern Baltic Sea under environmental change, ICES J. Mar. Sci., 77, 624–632, https://doi.org/10.1093/icesjms/fsz224, 2020.
Neumann, T., Eilola, K., Gustafsson, B., Müller-Karulis, B., Kuznetsov, I., Meier, H. E. M., and Savchuk, O. P.: Extremes of temperature, oxygen and blooms in the Baltic Sea in a changing climate, Ambio, 41, 574–585, https://doi.org/10.1007/s13280-012-0321-2, 2012.
Niiranen, S., Yletyinen, J., Tomczak, M. T., Blenckner, T., Hjerne, O., MacKenzie, B. R., Müller-Karulis, B., Neumann, T., and Meier, H. E. M.: Combined effects of global climate change and regional ecosystem drivers on an exploited marine food web, Glob. Change Biol., 19, 3327–3342, https://doi.org/10.1111/gcb.12309, 2013.
Norkko, J., Reed, D. C., Timmermann, K., Norkko, A., Gustafsson, B. G., Bonsdorff, E., Slomp, C. P., Carstensen, J., and Conley, D. J.: A welcome can of worms? Hypoxia mitigation by an invasive species, Glob. Change Biol., 18, 422–434, https://doi.org/10.1111/j.1365-2486.2011.02513.x, 2012.
Nydahl, A., Panigrahi, S., and Wikner, J.: Increased microbial activity in a warmer and wetter climate enhances the risk of coastal hypoxia, FEMS Microbiol. Ecol., 85, 338–347, https://doi.org/10.1111/1574-6941.12123, 2013.
Olli, K., Klais, R., Tamminen, T., Ptacnik, R., and Andersen, T.: Long term changes in the Baltic Sea phytoplankton community, Boreal Environ. Res., 16, 3–14, 2011.
Olofsson, M., Torstensson, A., Karlberg, M., Steinhoff, F. S., Dinasquet, J., Riemann, L., Chierici, M., and Wulff, A.: Limited response of a spring bloom community inoculated with filamentous cyanobacteria to elevated temperature and pCO2, Bot. Mar., 62, 3–16, https://doi.org/10.1515/bot-2018-0005, 2019.
Olofsson, M., Suikkanen, S., Kobos, J., Wasmund, N., and Karlson, B.: Basin-specific changes in filamentous cyanobacteria community composition across four decades in the Baltic Sea, Harmful Algae, 91, 12, https://doi.org/10.1016/j.hal.2019.101685, 2020.
Olsson, J., Tomczak, M. T., Ojaveer, H., Gårdmark, A., Pöllumae, A., Müller-Karulis, B., Ustups, D., Dinesen, G. E., Peltonen, H., Putnis, I., Szymanek, L., Simm, M., Heikinheimo, O., Gasyukov, P., Axe, P., and Bergström, L.: Temporal development of coastal ecosystems in the Baltic Sea over the past two decades, ICES J. Mar. Sci., 72, 2539–2548, https://doi.org/10.1093/icesjms/fsv143, 2015.
Omran, E.-S. E. and Negm, A. M.: Overview of the water bodies in the Baltic Sea countries, in: Water resources quality and management in the Baltic Sea countries, edited by: Negm, A. M., Zelenakova, M., and Kubiak-Wójcicka, K., Springer Water, Springer Nature Switzerland AG, Cham, 17–42, ISBN 978-3-030-39701-2, 2020.
Orio, A., Bergstrom, U., Florin, A. B., Sics, I., and Casini, M.: Long-term changes in spatial overlap between interacting cod and flounder in the Baltic Sea, Hydrobiologia, 847, 2541–2553, https://doi.org/10.1007/s10750-020-04272-4, 2020.
Orio, A., Heimbrand, Y., and Limburg, K.: Deoxygenation impacts on Baltic Sea cod: Dramatic declines in ecosystem services of an iconic keystone predator, Ambio, 51, 626–637, https://doi.org/10.1007/s13280-021-01572-4, 2021.
Otto, S. A., Kornilovs, G., Llope, M., and Möllmann, C.: Interactions among density, climate, and food web effects determine long-term life cycle dynamics of a key copepod, Mar. Ecol. Prog. Ser., 498, 73-U408, https://doi.org/10.3354/meps10613, 2014a.
Otto, S. A., Diekmann, R., Flinkman, J., Kornilovs, G., and Möllmann, C.: Habitat heterogeneity determines climate impact on zooplankton community structure and dynamics, PLoS ONE, 9, e90875, https://doi.org/10.1371/journal.pone.0090875, 2014b.
Paasche, Ø., Österblom, H., Neuenfeldt, S., Bonsdorff, E., Brander, K., Conley, D. J., Durant, J. M., Eikeset, A. M., Goksøyr, A., and Jónsson, S.: Connecting the seas of norden, Nat. Clim. Change, 5, 89, 2015.
Pajusalu, L., Martin, G., and Pöllumae, A.: Results of laboratory and field experiments of the direct effect of increasing CO2 on net primary production of macroalgal species in brackish-water ecosystems, P. Est. Acad. Sci., 62, 148–154, https://doi.org/10.3176/proc.2013.2.09, 2013.
Pajusalu, L., Martin, G., Pöllumae, A., Torn, K., and Paalme, T.: Direct effects of increased CO2 concentrations in seawater on the net primary production of charophytes in a shallow, coastal, brackish-water ecosystem, Boreal Environ. Res., 20, 413–422, 2015.
Pajusalu, L., Martin, G., Paalme, T., and Pöllumae, A.: The effect of CO2 enrichment on net photosynthesis of the red alga Furcellaria lumbricalis in a brackish water environment, Peerj, 4, e2505, https://doi.org/10.7717/peerj.2505, 2016.
Pansch, C., Nasrolahi, A., Appelhans, Y. S., and Wahl, M.: Impacts of ocean warming and acidification on the larval development of the barnacle Amphibalanus improvisus, J. Exp. Mar. Biol. Ecol., 420, 48–55, https://doi.org/10.1016/j.jembe.2012.03.023, 2012.
Pansch, C., Scotti, M., Barboza, F. R., Al-Janabi, B., Brakel, J., Briski, E., Bucholz, B., Franz, M., Ito, M., Paiva, F., Saha, M., Sawall, Y., Weinberger, F., and Wahl, M.: Heat waves and their significance for a temperate benthic community: A near-natural experimental approach, Glob. Change Biol., 24, 4357–4367, https://doi.org/10.1111/gcb.14282, 2018.
Paul, A. J., Sommer, U., Paul, C., and Riebesell, U.: Baltic Sea diazotrophic cyanobacterium is negatively affected by acidification and warming, Mar. Ecol. Prog. Ser., 598, 49–60, https://doi.org/10.3354/meps12632, 2018.
Paul, C., Matthiessen, B., and Sommer, U.: Warming, but not enhanced CO2 concentration, quantitatively and qualitatively affects phytoplankton biomass, Mar. Ecol. Prog. Ser., 528, 39–51, https://doi.org/10.3354/meps11264, 2015.
Paul, C., Sommer, U., Garzke, J., Moustaka-Gouni, M., Paul, A., and Matthiessen, B.: Effects of increased CO2 concentration on nutrient limited coastal summer plankton depend on temperature, Limnol. Oceanogr., 61, 853–868, https://doi.org/10.1002/lno.10256, 2016.
Pecuchet, L., Lindegren, M., Kortsch, S., Calkiewicz, J., Jurgensone, I., Margonski, P., Otto, S. A., Putnis, I., Strake, S., and Nordström, M. C.: Spatio-temporal dynamics of multi-trophic communities reveal ecosystem-wide functional reorganization, Ecography, 43, 197–208, https://doi.org/10.1111/ecog.04643, 2020.
Pekcan-Hekim, Z., Urho, L., Auvinen, H., Heikinheimo, O., Lappalainen, J., Raitaniemi, J., and Söderkultalahti, P.: Climate warming and pikeperch year-class catches in the Baltic Sea, Ambio, 40, 447–456, https://doi.org/10.1007/s13280-011-0143-7, 2011.
Pekcan-Hekim, Z., Gårdmark, A., Karlson, A. M. L., Kauppila, P., Bergenius, M., and Bergström, L.: The role of climate and fisheries on the temporal changes in the Bothnian Bay foodweb, ICES J. Mar. Sci., 73, 1739–1749, https://doi.org/10.1093/icesjms/fsw032, 2016.
Philippart, C. J. M., Anadon, R., Danovaro, R., Dippner, J. W., Drinkwater, K. F., Hawkins, S. J., Oguz, T., O'Sullivan, G., and Reid, P. C.: Impacts of climate change on European marine ecosystems: Observations, expectations and indicators, J. Exp. Mar. Biol. Ecol., 400, 52–69, https://doi.org/10.1016/j.jembe.2011.02.023, 2011.
Pihlainen, S., Zandersen, M., Hyytiäinen, K., Andersen, H. E., Bartosova, A., Gustafsson, B., Jabloun, M., McCrackin, M., Meier, H. M., and Olesen, J. E.: Impacts of changing society and climate on nutrient loading to the Baltic Sea, Sci. Total Environ., 731, 138935, https://doi.org/10.1016/j.scitotenv.2020.138935, 2020.
Puttonen, I., Mattila, J., Jonsson, P., Karlsson, O. M., Kohonen, T., Kotilainen, A., Lukkari, K., Malmaeus, J. M., and Rydin, E.: Distribution and estimated release of sediment phosphorus in the northern Baltic Sea archipelagos, Estuar. Coast. Shelf S., 145, 9–21, https://doi.org/10.1016/j.ecss.2014.04.010, 2014.
Puttonen, I., Kohonen, T., and Mattila, J.: Factors controlling phosphorus release from sediments in coastal archipelago areas, Mar. Pollut. Bull., 108, 77–86, https://doi.org/10.1016/j.marpolbul.2016.04.059, 2016.
Queiros, A. M., Talbot, E., Beaumont, N. J., Somerfield, P. J., Kay, S., Pascoe, C., Dedman, S., Fernandes, J. A., Jueterbock, A., Miller, P. I., Sailley, S. F., Sara, G., Carr, L. M., Austen, M. C., Widdicombe, S., Rilov, G., Levin, L. A., Hull, S. C., Walmsley, S. F., and Aonghusa, C. N.: Bright spots as climate-smart marine spatial planning tools for conservation and blue growth, Glob. Change Biol., 27, 5514–5531, https://doi.org/10.1111/gcb.15827, 2021.
Reusch, T. B. H., Dierking, J., Andersson, H. C., Bonsdorff, E., Carstensen, J., Casini, M., Czajkowski, M., Hasler, B., Hinsby, K., Hyytiainen, K., Johannesson, K., Jomaa, S., Jormalainen, V., Kuosa, H., Kurland, S., Laikre, L., MacKenzie, B. R., Margonski, P., Melzner, F., Oesterwind, D., Ojaveer, H., Refsgaard, J. C., Sandström, A., Schwarz, G., Tonderski, K., Winder, M., and Zandersen, M.: The Baltic Sea as a time machine for the future coastal ocean, Science Advances, 4, eaar8195, https://doi.org/10.1126/sciadv.aar8195, 2018.
Rinne, H. and Salovius-Laurén, S.: The status of brown macroalgae Fucus spp. and its relation to environmental variation in the Finnish marine area, northern Baltic Sea, Ambio, 49, 118–129, https://doi.org/10.1007/s13280-019-01175-0, 2020.
Röhr, M. E., Boström, C., Canal-Vergés, P., and Holmer, M.: Blue carbon stocks in Baltic Sea eelgrass (Zostera marina) meadows, Biogeosciences, 13, 6139–6153, https://doi.org/10.5194/bg-13-6139-2016, 2016.
Rolff, C. and Elfwing, T.: Increasing nitrogen limitation in the Bothnian Sea, potentially caused by inflow of phosphate-rich water from the Baltic Proper, Ambio, 44, 601–611, https://doi.org/10.1007/s13280-015-0675-3, 2015.
Rothäusler, E., Rugiu, L., and Jormalainen, V.: Forecast climate change conditions sustain growth and physiology but hamper reproduction in range-margin populations of a foundation rockweed species, Mar. Environ. Res., 141, 205–213, https://doi.org/10.1016/j.marenvres.2018.09.014, 2018.
Rothäusler, E., Uebermuth, C., Haavisto, F., and Jormalainen, V.: Living on the edge: Gamete release and subsequent fertilisation in Fucus vesiculosus (Phaeophyceae) are weakened by climate change-forced hyposaline conditions, Phycologia, 58, 111–114, https://doi.org/10.1080/00318884.2018.1524246, 2019.
Rousi, H., Laine, A. O., Peltonen, H., Kangas, P., Andersin, A. B., Rissanen, J., Sandberg-Kilpi, E., and Bonsdorff, E.: Long-term changes in coastal zoobenthos in the northern Baltic Sea: the role of abiotic environmental factors, ICES J. Mar. Sci., 70, 440–451, https://doi.org/10.1093/icesjms/fss197, 2013.
Rousi, H. E. J., Korpinen, S., and Bonsdorff, E.: Brackish-water benthic fauna under fluctuating environmental conditions: the role of eutrophication, hypoxia and global change, Front. Mar. Sci., 6, 464, 2019.
Rugiu, L., Manninen, I., Rothäusler, E., and Jormalainen, V.: Tolerance and potential for adaptation of a Baltic Sea rockweed under predicted climate change conditions, Mar. Environ. Res., 134, 76–84, https://doi.org/10.1016/j.marenvres.2017.12.016, 2018a.
Rugiu, L., Manninen, I., Rothäusler, E., and Jormalainen, V.: Tolerance to climate change of the clonally reproducing endemic Baltic seaweed, Fucus radicans: is phenotypic plasticity enough?, J. Phycol., 54, 888–898, https://doi.org/10.1111/jpy.12796, 2018b.
Rugiu, L., Manninen, I., Sjoroos, J., and Jormalainen, V.: Variations in tolerance to climate change in a key littoral herbivore, Mar. Biol., 165, 1–11, https://doi.org/10.1007/s00227-017-3275-x, 2018c.
Ryabchenko, V. A., Karlin, L. N., Isaev, A. V., Vankevich, R. E., Eremina, T. R., Molchanov, M. S., and Savchuk, O. P.: Model estimates of the eutrophication of the Baltic Sea in the contemporary and future climate, Oceanology, 56, 36–45, https://doi.org/10.1134/s0001437016010161, 2016.
Saha, M., Barboza, F. R., Somerfield, P. J., Al-Janabi, B., Beck, M., Brakel, J., Ito, M., Pansch, C., Nascimento-Schulze, J. C., Thor, S. J., Weinberger, F., and Sawall, Y.: Response of foundation macrophytes to near-natural simulated marine heatwaves, Glob. Change Biol., 26, 417–430, https://doi.org/10.1111/gcb.14801, 2020.
Sahla, M., Tolvanen, H., Ruuskanen, A., and Kurvinen, L.: Assessing long term change of Fucus spp. communities in the northern Baltic Sea using monitoring data and spatial modeling, Estuar. Coast. Shelf S., 245, 107023, https://doi.org/10.1016/j.ecss.2020.107023, 2020.
Salo, T., Mattila, J., and Eklöf, J.: Long-term warming affects ecosystem functioning through species turnover and intraspecific trait variation, Oikos, 129, 283–295, https://doi.org/10.1111/oik.06698, 2020.
Saraiva, S., Meier, H. E. M., Andersson, H., Höglund, A., Dieterich, C., Gröger, M., Hordoir, R., and Eilola, K.: Baltic Sea ecosystem response to various nutrient load scenarios in present and future climates, Clim. Dynam., 52, 3369–3387, https://doi.org/10.1007/s00382-018-4330-0, 2018.
Saraiva, S., Meier, H. E. M., Andersson, H., Höglund, A., Dieterich, C., Gröger, M., Hordoir, R., and Eilola, K.: Uncertainties in projections of the Baltic Sea ecosystem driven by an ensemble of Global Climate Models, Front. Earth Sci., 6, 244, https://doi.org/10.3389/feart.2018.00244, 2019.
Sawall, Y., Ito, M., and Pansch, C.: Chronically elevated sea surface temperatures revealed high susceptibility of the eelgrass Zostera marina to winter and spring warming, Limnol. Oceanogr., 66, 4112–4124, https://doi.org/10.1002/lno.11947, 2021.
Schmidt, K., Birchill, A. J., Atkinson, A., Brewin, R. J. W., Clark, J. R., Hickman, A. E., Johns, D. G., Lohan, M. C., Milne, A., Pardo, S., Polimene, L., Smyth, T. J., Tarran, G. A., Widdicombe, C. E., Woodward, E. M. S., and Ussher, S. J.: Increasing picocyanobacteria success in shelf waters contributes to long-term food web degradation, Glob. Change Biol., 26, 5574–5587, https://doi.org/10.1111/gcb.15161, 2020.
Sherman, K., Belkin, I. M., Friedland, K. D., O'Reilly, J., and Hyde, K.: Accelerated warming and emergent trends in fisheries biomass yields of the world's Large Marine Ecosystems, Ambio, 38, 215–224, https://doi.org/10.1579/0044-7447-38.4.215, 2009.
Skogen, M. D., Eilola, K., Hansen, J. L. S., Meier, H. E. M., Molchanov, M. S., and Ryabchenko, V. A.: Eutrophication status of the North Sea, Skagerrak, Kattegat and the Baltic Sea in present and future climates: A model study, J. Marine Syst., 132, 174–184, https://doi.org/10.1016/j.jmarsys.2014.02.004, 2014.
Snickars, M., Weigel, B., and Bonsdorff, E.: Impact of eutrophication and climate change on fish and zoobenthos in coastal waters of the Baltic Sea, Mar. Biol., 162, 141–151, https://doi.org/10.1007/s00227-014-2579-3, 2015.
Sommer, U. and Lewandowska, A.: Climate change and the phytoplankton spring bloom: warming and overwintering zooplankton have similar effects on phytoplankton, Glob. Change Biol., 17, 154–162, https://doi.org/10.1111/j.1365-2486.2010.02182.x, 2011.
Sommer, U., Aberle, N., Lengfellner, K., and Lewandowska, A.: The Baltic Sea spring phytoplankton bloom in a changing climate: an experimental approach, Mar. Biol., 159, 2479–2490, https://doi.org/10.1007/s00227-012-1897-6, 2012.
Sommer, U., Paul, C., and Moustaka-Gouni, M.: Warming and ocean acidification effects on phytoplankton – From species shifts to size shifts within species in a mesocosm experiment, PLoS ONE, 10, e0125239, https://doi.org/10.1371/journal.pone.0125239, 2015.
Sopanen, S., Setälä, O., Piiparinen, J., Erler, K., and Kremp, A.: The toxic dinoflagellate Alexandrium ostenfeldii promotes incapacitation of the calanoid copepods Eurytemora affinis and Acartia bifilosa from the northern Baltic Sea, J. Plankton Res., 33, 1564–1573, https://doi.org/10.1093/plankt/fbr052, 2011.
Sparrevohn, C. R., Lindegren, M., and Mackenzie, B. R.: Climate-induced response of commercially important flatfish species during the 20th century, Fish. Oceanogr., 22, 400–408, https://doi.org/10.1111/fog.12030, 2013.
Spilling, K., Olli, K., Lehtoranta, J., Kremp, A., Tedesco, L., Tamelander, T., Klais, R., Peltonen, H., and Tamminen, T.: Shifting diatom–dinoflagellate dominance during spring bloom in the Baltic Sea and its potential effects on biogeochemical cycling, Front. Mar. Sci., 5, 327, https://doi.org/10.3389/fmars.2018.00327, 2018.
Stenseth, N. C., Payne, M. R., Bonsdorff, E., Dankel, D. J., Durant, J. M., Anderson, L. G., Armstrong, C. W., Blenckner, T., Brakstad, A., Dupont, S., Eikeset, A. M., Goksøyr, A., Jónsson, S., Kuparinen, A., Vage, K., Österblom, H., and Paasche, O.: Attuning to a changing ocean, P. Natl. Acad. Sci. USA, 117, 20363–20371, https://doi.org/10.1073/pnas.1915352117, 2020.
Stiasny, M. H., Mittermayer, F. H., Sswat, M., Voss, R., Jutfelt, F., Chierici, M., Puvanendran, V., Mortensen, A., Reusch, T. B. H., and Clemmesen, C.: Ocean acidification effects on Atlantic cod larval survival and recruitment to the fished population, PLoS ONE, 11, e0155448, https://doi.org/10.1371/journal.pone.0155448, 2016.
Stigebrandt, A. and Anderson, A.: The eutrophication of the Baltic Sea has been boosted and perpetuated by a major internal phosphorus source, Front. Mar. Sci., 7, 14, https://doi.org/10.3389/fmars.2020.572994, 2020.
Stigebrandt, A., Rahm, L., Viktorsson, L., Ödalen, M., Hall, P. O. J., and Liljebladh, B.: A new phosphorus paradigm for the Baltic proper, Ambio, 43, 634–643, https://doi.org/10.1007/s13280-013-0441-3, 2014.
Strååt, K. D., Morth, C. M., and Undeman, E.: Future export of particulate and dissolved organic carbon from land to coastal zones of the Baltic Sea, J. Marine Syst., 177, 8–20, https://doi.org/10.1016/j.jmarsys.2017.09.002, 2018.
Suikkanen, S., Pulina, S., Engström-Öst, J., Lehtiniemi, M., Lehtinen, S., and Brutemark, A.: Climate change and eutrophication induced shifts in northern summer plankton communities, PLoS ONE, 8, e66475, https://doi.org/10.1371/journal.pone.0066475, 2013.
Svensson, F., Karlsson, E., Gårdmark, A., Olsson, J., Adill, A., Zie, J., Snoeijs, P., and Eklöf, J. S.: In situ warming strengthens trophic cascades in a coastal food web, Oikos, 126, 1150–1161, https://doi.org/10.1111/oik.03773, 2017.
Takolander, A., Cabeza, M., and Leskinen, E.: Climate change can cause complex responses in Baltic Sea macroalgae: A systematic review, J. Sea Res., 123, 16–29, https://doi.org/10.1016/j.seares.2017.03.007, 2017a.
Takolander, A., Leskinen, E., and Cabeza, M.: Synergistic effects of extreme temperature and low salinity on foundational macroalga Fucus vesiculosus in the northern Baltic Sea, J. Exp. Mar. Biol. Ecol., 495, 110–118, https://doi.org/10.1016/j.jembe.2017.07.001, 2017b.
Tedesco, L., Piroddi, C., Kamari, M., and Lynam, C.: Capabilities of Baltic Sea models to assess environmental status for marine biodiversity, Mar. Policy, 70, 1–12, https://doi.org/10.1016/j.marpol.2016.04.021, 2016.
Tedesco, L., Miettunen, E., An, B. W., Haapala, J., and Kaartokallio, H.: Long-term mesoscale variability of modelled sea-ice primary production in the northern Baltic Sea, Elementa-Sci. Anthrop., 5, 26, https://doi.org/10.1525/elementa.223, 2017.
The BACC Author Team: Assessment of climate change for the Baltic Sea basin, Regional Climate Studies, Springer-Verlag, Berlin, Heidelberg, 473ṗp., ISBN 978-3-540-72786-6, 2008.
The BACC II Author Team: Second Assessment of Climate Change for the Baltic Sea Basin, Regional Climate Studies, Springer, Cham, Heidelberg, New York, Dordrecht, London, 501 pp., https://doi.org/10.1007/978-3-319-16006-1, 2015.
Thomas, D. N., Kaartokallio, H., Tedesco, L., Majaneva, M., Piiparinen, J., Eronen-Rasimus, E., Rintala, J.-M., Kuosa, H., Blomster, J., and Vainio, J.: Life associated with Baltic Sea ice, in: Biological oceanography of the Baltic Sea, Springer, 333–357, https://doi.org/10.1007/978-94-007-0668-2, 2017.
Tilman, D.: Functional diversity, Encyclopedia of biodiversity, 3, 109–120, https://doi.org/10.1006/rwbd.1999.0154, 2001.
Timmermann, K., Norkko, J., Janas, U., Norkko, A., Gustafsson, B. G., and Bonsdorff, E.: Modelling macrofaunal biomass in relation to hypoxia and nutrient loading, J. Marine Syst., 105, 60–69, https://doi.org/10.1016/j.jmarsys.2012.06.001, 2012.
Tomczak, M. T., Müller-Karulis, B., Blenckner, T., Ehrnsten, E., Eero, M., Gustafsson, B., Norkko, A., Otto, S. A., Timmermann, K., and Humborg, C.: Reference state, structure, regime shifts, and regulatory drivers in a coastal sea over the last century: The Central Baltic Sea case, Limnol. Oceanogr., 67, S266–S284, https://doi.org/10.1002/lno.11975, 2021.
Torn, K., Peterson, A., and Herkül, K.: Predicting the impact of climate change on the distribution of the key habitat-forming species in the NE Baltic Sea, J. Coast. Res., 177–181, https://doi.org/10.2112/si95-035.1, 2020.
Törnroos, A., Pecuchet, L., Olsson, J., Gårdmark, A., Blomqvist, M., Lindegren, M., and Bonsdorff, E.: Four decades of functional community change reveals gradual trends and low interlinkage across trophic groups in a large marine ecosystem, Glob. Change Biol., 25, 1235–1246, https://doi.org/10.1111/gcb.14552, 2019.
Turja, R., Hoher, N., Snoeijs, P., Barsiene, J., Butrimaviciene, L., Kuznetsova, T., Kholodkevich, S. V., Devier, M. H., Budzinski, H., and Lehtonen, K. K.: A multibiomarker approach to the assessment of pollution impacts in two Baltic Sea coastal areas in Sweden using caged mussels (Mytilus trossulus), Sci. Total Environ., 473, 398–409, https://doi.org/10.1016/j.scitotenv.2013.12.038, 2014.
Turja, R., Lehtonen, K. K., Meierjohann, A., Brozinski, J. M., Vahtera, E., Soirinsuo, A., Sokolov, A., Snoeijs, P., Budzinski, H., Devier, M. H., Peluhet, L., Pääkkönen, J.-P., Viitasalo, M., and Kronberg, L.: The mussel caging approach in assessing biological effects of wastewater treatment plant discharges in the Gulf of Finland (Baltic Sea), Mar. Pollut. Bull., 97, 135–149, https://doi.org/10.1016/j.marpolbul.2015.06.024, 2015.
Vahtera, E., Conley, D. J., Gustafsson, B. G., Kuosa, H., Pitkänen, H., Savchuk, O. P., Tamminen, T., Viitasalo, M., Voss, M., Wasmund, N., and Wulff, F.: Internal ecosystem feedbacks enhance nitrogen-fixing cyanobacteria blooms and complicate management in the Baltic Sea, Ambio, 36, 186–194, https://doi.org/10.1579/0044-7447(2007)36[186:iefenc]2.0.co;2, 2007.
Vehmaa, A., Hogfors, H., Gorokhova, E., Brutemark, A., Holmborn, T., and Engström-Öst, J.: Projected marine climate change: effects on copepod oxidative status and reproduction, Ecol. Evol., 3, 4548–4557, https://doi.org/10.1002/ece3.839, 2013.
Vehmaa, A., Almén, A.-K., Brutemark, A., Paul, A., Riebesell, U., Furuhagen, S., and Engström-Öst, J.: Ocean acidification challenges copepod phenotypic plasticity, Biogeosciences, 13, 6171–6182, https://doi.org/10.5194/bg-13-6171-2016, 2016.
Vigouroux, G., Kari, E., Beltrán-Abaunza, J. M., Uotila, P., Yuan, D., and Destouni, G.: Trend correlations for coastal eutrophication and its main local and whole-sea drivers–Application to the Baltic Sea, Sci. Total Environ., 779, 146367, https://doi.org/10.1016/j.scitotenv.2021.146367, 2021.
Viitasalo, M., Blenckner, T., Gårdmark, A., Kautsky, L., Kaartokallio, H., Kuosa, H., Lindegren, M., Norkko, A., Olli, K., and Wikner, J.: Environmental impacts – Marine ecosystems, in: Second Assessment of Climate Change for the Baltic Sea basin, edited by: The BACC II Author Team, Springer, Berlin, Heidelberg, 363–380, ISBN 978-3-319-16006-1, 2015.
Villnäs, A., Norkko, A., and Lehtonen, K. K.: Multi-level responses of Macoma balthica to recurring hypoxic disturbance, J. Exp. Mar. Biol. Ecol., 510, 64–72, https://doi.org/10.1016/j.jembe.2018.10.005, 2019.
Virtanen, E. A., Viitasalo, M., Lappalainen, J., and Moilanen, A.: Evaluation, gap analysis, and potential expansion of the Finnish marine protected area network, Front. Mar. Sci., 5, 402, 2018.
Virtanen, E. A., Norkko, A., Nyström Sandman, A., and Viitasalo, M.: Identifying areas prone to coastal hypoxia – the role of topography, Biogeosciences, 16, 3183–3195, https://doi.org/10.5194/bg-16-3183-2019, 2019.
Virtanen, E. A., Moilanen, A., and Viitasalo, M.: Marine connectivity in spatial conservation planning: analogues from the terrestrial realm, Landscape Ecol., 35, 1021–1034, https://doi.org/10.1007/s10980-020-00997-8, 2020.
Voss, M., Larsen, B., Leivuori, M., and Vallius, H.: Stable isotope signals of eutrophication in Baltic Sea sediments, J. Marine Syst., 25, 287–298, https://doi.org/10.1016/s0924-7963(00)00022-1, 2000.
Voss, M., Dippner, J. W., Humborg, C., Hurdler, J., Korth, F., Neumann, T., Schernewski, G., and Venohr, M.: History and scenarios of future development of Baltic Sea eutrophication, Estuar. Coast. Shelf S., 92, 307–322, https://doi.org/10.1016/j.ecss.2010.12.037, 2011.
Voss, R., Hinrichsen, H. H., Quaas, M. F., Schmidt, J. O., and Tahvonen, O.: Temperature change and Baltic sprat: from observations to ecological-economic modelling, ICES J. Mar. Sci., 68, 1244–1256, https://doi.org/10.1093/icesjms/fsr063, 2011.
Voss, R., Petereit, C., Schmidt, J. O., Lehmann, A., Makarchouk, A., and Hinrichsen, H. H.: The spatial dimension of climate-driven temperature change in the Baltic Sea and its implication for cod and sprat early life stage survival, J. Marine Syst., 100, 1–8, https://doi.org/10.1016/j.jmarsys.2012.03.009, 2012.
Vuorinen, I., Hänninen, J., Rajasilta, M., Laine, P., Eklund, J., Montesino-Pouzols, F., Corona, F., Junker, K., Meier, H. E. M., and Dippner, J. W.: Scenario simulations of future salinity and ecological consequences in the Baltic Sea and adjacent North Sea areas – Implications for environmental monitoring, Ecol. Indic., 53, 294–294, https://doi.org/10.1016/j.ecolind.2015.01.030, 2015.
Wahl, M., Werner, F. J., Buchholz, B., Raddatz, S., Graiff, A., Matthiessen, B., Karsten, U., Hiebenthal, C., Hamer, J., Ito, M., Gulzow, E., Rilov, G., and Guy-Haim, T.: Season affects strength and direction of the interactive impacts of ocean warming and biotic stress in a coastal seaweed ecosystem, Limnol. Oceanogr., 65, 807–827, https://doi.org/10.1002/lno.11350, 2019.
Wahl, M., Barboza, F. R., Buchholz, B., Dobretsov, S., Guy-Haim, T., Rilov, G., Schuett, R., Wolf, F., Vajedsamiei, J., Yazdanpanah, M., and Pansch, C.: Pulsed pressure: Fluctuating impacts of multifactorial environmental change on a temperate macroalgal community, Limnol. Oceanogr., 66, 4210–4226, https://doi.org/10.1002/lno.11954, 2021.
Wåhlström, I., Höglund, A., Almroth-Rosell, E., MacKenzie, B. R., Gröger, M., Eilola, K., Plikshs, M., and Andersson, H. C.: Combined climate change and nutrient load impacts on future habitats and eutrophication indicators in a eutrophic coastal sea, Limnol. Oceanogr., 65, 2170–2187, 2020.
Wasmund, N., Tuimala, J., Suikkanen, S., Vandepitte, L., and Kraberg, A.: Long-term trends in phytoplankton composition in the western and central Baltic Sea, J. Marine Syst., 87, 145–159, https://doi.org/10.1016/j.jmarsys.2011.03.010, 2011.
Wasmund, N., Nausch, G., Gerth, M., Busch, S., Burmeister, C., Hansen, R., and Sadkowiak, B.: Extension of the growing season of phytoplankton in the western Baltic Sea in response to climate change, Mar. Ecol. Prog. Ser., 622, 1–16, https://doi.org/10.3354/meps12994, 2019.
Weber, S. C., Loick-Wilde, N., Montoya, J. P., Bach, M., Hai, D. N., Subramaniam, A., Liskow, I., Lam, N. N., Wodarg, D., and Voss, M.: Environmental Regulation of the Nitrogen Supply, Mean Trophic Position, and Trophic Enrichment of Mesozooplankton in the Mekong River Plume and Southern South China Sea, J. Geophys. Res.: Oceans, 126, 19, https://doi.org/10.1029/2020jc017110, 2021.
Weigel, B., Andersson, H. C., Meier, H. E. M., Blenckner, T., Snickars, M., and Bonsdorff, E.: Long-term progression and drivers of coastal zoobenthos in a changing system, Mar. Ecol. Prog. Ser., 528, 141–159, https://doi.org/10.3354/meps11279, 2015.
Werner, F. J. and Matthiessen, B.: Warming has stronger direct than indirect effects on benthic microalgae in a seaweed system in spring, Mar. Biol., 164, 1–10, https://doi.org/10.1007/s00227-017-3109-x, 2017.
Werner, F. J., Graiff, A., and Matthiessen, B.: Temperature effects on seaweed-sustaining top-down control vary with season, Oecologia, 180, 889–901, https://doi.org/10.1007/s00442-015-3489-x, 2016.
Wikner, J. and Andersson, A.: Increased freshwater discharge shifts the trophic balance in the coastal zone of the northern Baltic Sea, Glob. Change Biol., 18, 2509–2519, https://doi.org/10.1111/j.1365-2486.2012.02718.x, 2012.
Wood, H. L., Sköld, H. N., and Eriksson, S. P.: Health and population-dependent effects of ocean acidification on the marine isopod Idotea balthica, Mar. Biol., 161, 2423–2431, https://doi.org/10.1007/s00227-014-2518-3, 2014.
Woods, P., Macdonald, J., Bárðarson, H., Bonanomi, S., Boonstra, W., Cornell, G., Cripps, G., Danielsen, R., Färber, L., and Ferreira, A.: A review of adaptation options in fisheries management to support resilience and transition under socio-ecological change, ICES J. Mar. Sci., 79, 463–479, https://doi.org/10.1093/icesjms/fsab146, 2021.
Wulff, A., Karlberg, M., Olofsson, M., Torstensson, A., Riemann, L., Steinhoff, F. S., Mohlin, M., Ekstrand, N., and Chierici, M.: Ocean acidification and desalination: climate-driven change in a Baltic Sea summer microplanktonic community, Mar. Biol., 165, 1–15, https://doi.org/10.1007/s00227-018-3321-3, 2018.
Yletyinen, J., Bodin, O., Weigel, B., Nordström, M. C., Bonsdorff, E., and Blenckner, T.: Regime shifts in marine communities: a complex systems perspective on food web dynamics, P. Roy. Soc. B-Biol. Sci., 283, 20152569, https://doi.org/10.1098/rspb.2015.2569, 2016.
- Abstract
- Introduction
- Definitions
- Review methods
- Effects on species and communities
- Climate change and ecosystem structure and function
- Knowledge gaps
- Conclusions
- Data availability
- Author contributions
- Competing interests
- Disclaimer
- Special issue statement
- Acknowledgements
- Financial support
- Review statement
- References
- Abstract
- Introduction
- Definitions
- Review methods
- Effects on species and communities
- Climate change and ecosystem structure and function
- Knowledge gaps
- Conclusions
- Data availability
- Author contributions
- Competing interests
- Disclaimer
- Special issue statement
- Acknowledgements
- Financial support
- Review statement
- References