ESD Ideas: Photoelectrochemical carbon removal as negative emission technology
The pace of the transition to a low-carbon economy – especially in the fuels sector – is not high enough to achieve the 2 ∘C target limit for global warming by only cutting emissions. Most political roadmaps to tackle global warming implicitly rely on the timely availability of mature negative emission technologies, which actively invest energy to remove CO2 from the atmosphere and store it permanently. The models used as a basis for decarbonization policies typically assume an implementation of such large-scale negative emission technologies starting around the year 2030, ramped up to cause net negative emissions in the second half of the century and balancing earlier CO2 release. On average, a contribution of −10 Gt CO2 yr−1 is expected by 2050 (Anderson and Peters, 2016). A viable approach for negative emissions should (i) rely on a scalable and sustainable source of energy (solar), (ii) result in a safely storable product, (iii) be highly efficient in terms of water and energy use, to reduce the required land area and competition with water and food demands of a growing world population, and (iv) feature large-scale feasibility and affordability.
Processes for the extraction of CO2 from the atmosphere are energy-intensive. This energy has to be supplied by low- or zero-carbon sources. At present, primarily direct air capture (followed by geologic injection) and biomass production are explored and there is an active discussion on costs and scalability of the various technologies (see Smith et al., 2016, and references cited therein). Renewably driven direct air capture is believed to be expensive and has not yet demonstrated scalability. Therefore, the currently most feasible option appears to be the use of natural photosynthesis to generate biomass through afforestation or ocean fertilization (Smith et al., 2016). Grown plants are then permanently stored, building a new stock of fossil fuels. Alternatively, the plant material can be combusted with carbon capture and storage to act as a low-carbon fuel. However, the efficiency of natural photosynthesis drops at high light conditions and because a significant fraction of the energy is used for the metabolism (Melis, 2012), the storage of solar energy in biomass is limited to 2 %–3 % efficiency. Therefore large areas of agricultural land would be required for the achievement of the negative emission goals: the removal of 1 Gt CO2 yr−1 can demand more than 1 million square kilometres, the combined area of Germany and France (Smith et al., 2016). There is an ongoing discussion of whether scaling biomass production to the required 10 Gt CO2 yr−1 is at all compatible with planetary constraints (Heck et al., 2018).
We suggest to employ photoelectrochemical CO2 reduction, also called artificial photosynthesis, to this end. As in its natural counterpart occurring in plants, photons in the artificial photosynthesis process excite charge carriers, which then reduce (and oxidize) reactants in a liquid electrolyte to solar fuels. The photon energy is only briefly converted to electron energy and then stored in molecular bonds. Light is absorbed in synthetic materials such as semiconductors or dyes and the chemical conversion typically takes place at (co)catalysts at the interface between electrolyte and light absorber. We primarily focus on tightly integrated photoelectrochemical systems, where the absorber is immersed into the electrolyte. While this approach imposes restrictions on the light absorber design, the tight integration also promises cost benefits (Kirner et al., 2016).
Artificial photosynthesis already delivers 5-fold higher efficiencies than natural photosynthesis, as 13 % for CO2 reduction and 19 % for solar water splitting have recently been demonstrated (Cheng et al., 2018; Schreier et al., 2017), more than half of the theoretical limits. Using solar fuels, either hydrogen or carbon-based from CO2 reduction, would cut greenhouse gas emissions. However, while the combustion product of hydrogen is water, using renewably generated carbon-based fuels releases the captured greenhouse gas back into the atmosphere. Recapturing the CO2 from the atmosphere would be energy-intensive and hereby lower the overall carbon reduction efficiency, which is why solar-energy-driven water splitting may be the preferable fuel, eliminating carbon completely from the energy system (Parkinson, 2016). Photoelectrochemical CO2 reduction could, therefore, be better placed to generate carbon-rich products that can safely and permanently store carbon extracted from the atmosphere. The electrochemical reactions have to be chosen to generate products that can be stored safely below-ground over thousands of years. Liquids or solids appear preferable, as gaseous products could leak back to the atmosphere, depending on the trapping mechanism (Damen et al., 2006). The handling of a solid product in an efficient flow-cell reactor is not practical. Although a large variety of products is in principle feasible, the production of carbon-rich liquids, such as alcohols or (fatty) acids, appears most promising. These could be stored in underground reservoirs such as depleted oil fields, be sequestered in the form of organic minerals, and used as precursors for organic construction materials.
Any competitive artificial approach should provide a significantly higher turnover than natural photosynthesis. To assess the technologies, their efficiency for carbon removal has to be estimated and compared. The typically used solar-to-fuel efficiency (May et al., 2017) is not suitable, as it only describes the relative fraction of incident solar radiation that is converted to chemical energy. Instead, negative emission technologies based on solar energy are better assessed by the solar-to-carbon (STC) efficiency, which we define as the ratio of converted CO2 molecules to the incoming photon flux (Appendix A).
Our calculations in the following were performed under the – highly idealized – assumption that the overpotential is dominated by the oxygen evolution reaction for a very good catalyst, which can be justified for water splitting. CO2 reduction with the currently available catalysts, on the other hand, is associated with significantly higher overpotentials. The direct impact of catalysis performance on achievable efficiencies can be seen in Fig. 1a and b, where obtainable STC efficiencies and resulting module areas are plotted as a function of Tafel slope and exchange current density.
Artificial solar energy conversion does – unlike natural photosynthesis – not suffer from an efficiency decrease due to high light conditions, as beneficial effects of light concentration on the solar cell and higher temperatures on catalysis can overcompensate the detrimental effect of temperature on the absorber. Hence, near-equatorial regions with high solar irradiation are viable target areas for its deployment. Under the assumption of 3500 kWh m−2 available per year for a two-axis tracker in the Sahara desert region (Amillo et al., 2014), we can estimate the required module area for the 2050 negative emission target of 10 Gt CO2 yr−1. At a maximum STC efficiency of ca. 19 % (for formic acid, see Appendix A), this would be approximately 13 500 km2. Under the assumption that for a mature technology the overall system efficiency is half of the theoretical efficiency, this translates to an areal requirement of about 27 000 km2 (Fig. 1c). The typical space factor for tracking photovoltaics of 0.2 (Araki et al., 2016) finally leads to a land footprint of ca. 135 000 km2. Other desert areas such as the Gobi desert, or the Thar Desert in north-western India, would also be interesting regions. In areas such as central Europe, a lower irradiance translates to larger footprints (Fig. 1d). The scale of such an effort, if one tried to realize it in a single project, would be considerable. However, it could be realized alongside with biomass approaches in other world regions, as it does not rely on agriculturally usable land. With the 2 ∘C target, there is a truly global incentive to realize such an undertaking. Especially if spread over several projects, the economic added value would be created in the regions that suffer most under global warming.
Carbon removal by artificial photosynthesis is water-efficient, compared to its natural counterpart, as water is only used as chemical precursor and not evaporated from the closed system. Considering formic acid a product to be stored, and the target of 10 Gt CO2 yr−1 to be removed, the water demand is about 4.1 Gt yr−1. This would be a substantial amount in dry regions. Desalination of seawater would be possible, albeit energetically inconvenient. However, the direct use of seawater was already demonstrated for electrochemical hydrogen production (Fukuzumi et al., 2017) and might therefore also be possible for CO2 reduction. Another challenge is that high-efficiency carbon sinks concentrated in large-scale facilities could, in principle, suffer from mass transport limitations of dilute CO2 in the atmosphere. This could be alleviated by selecting sites with high atmospheric convection rates, by spacing facilities sufficiently widely apart, or to combine them with solar updraft towers for electricity generation.
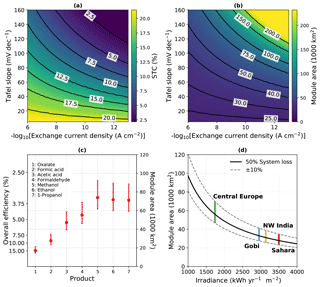
Figure 1Theoretical efficiency limits and module area for the −10 Gt CO2 yr−1 scenario. (a) STC efficiency limit of a dual-junction absorber for formic acid (without system loss) as a function of exchange current density and Tafel slope. (b) Resulting module area at Sahara irradiance and 50 % system loss. (c) STC efficiency and module area required under Sahara irradiance for a selection of products at 50 % system loss. Error bars indicate 40 % and 60 % loss, respectively. (d) Module area for formic acid production over the yearly irradiance at 50 % (solid line), as well as 40 % and 60 % (dashed lines) system loss. Vertical lines mark typical irradiances accessible to a two-axis tracker.
Requirements for the safe storage will vary significantly with the choice of the sink product. Formic acid would certainly be problematic due to its corrosiveness, also in the case of spilling events. Acetic acid and the alcohols are inflammable at high concentrations and would have to be diluted with water, increasing the water and volume footprint. For oxalate, the sink product with the highest STC efficiency, mineral trapping by reaction with a suitable calcium source, such as calcium chloride, to the stable mineral whewellite could be anticipated. The 10 Gt CO2 goal would result in roughly 17 Gt of the mineral. As a solid product, this – or other organic minerals – would not require underground injection and hence at this stage appears to be the most attractive option, with similar requirements as biomass storage. However, post-processing will increase the energy footprint and hereby also the costs.
In principle, electrochemical reduction of CO2 would also be possible using photovoltaics or wind power to first generate electricity, and then drive electrolysis and the chemical conversion. This introduces the intermediate step of converting solar to electrical energy. For the scales required, it appears that the potential of solar energy will, unlike wind, not be a limiting factor (Kleidon et al., 2016). Hybrid approaches, where inorganic solar cells are combined with bacteria, are also possible (Liu et al., 2015), but efficiencies are currently low and it is unclear how the drop in production rate under high illumination conditions can be overcome.
Artificial photosynthesis in the form of CO2 reduction represents consequently an interesting technological option for negative emissions due to its high efficiency. This would greatly reduce land use for the anticipated 2050 negative emission target compared to so far considered – mainly biomass-based – technologies. The installation of the required minimum module area of about 30 000 km2 would, however, still be an enormous undertaking. While we estimate the costs for photoelectrochemical CO2 conversion to the sink product to roughly EUR 65 per tonne (see Appendix B), we emphasize that the development stage of highly efficient photoelectrochemical CO2 conversion does not yet allow a robust estimate of the costs, rendering this value rather speculative. Furthermore, some of the anticipated sink products have an economic value as energy carriers and therefore require the creation of incentives to actually sequester and not combust the product. Physical feasibility and technological challenges can, however, already be anticipated. The greatest challenges to overcome with regards to the application are, for now, to develop and implement systems that are stable under operating conditions, as well as the derivation of earth-abundant, efficient catalysts (Cheng et al., 2018; Schreier et al., 2017).