ESD Ideas: Why are glaciations slower than deglaciations?
The Earth's climate during the Quaternary is dominated by short warm interglacials and longer cold glaciations paced by external forcings such as changes in insolation. Although not observed in the solar radiation changes, the time series of the cycles display asymmetry since transitions to full glacial conditions are slower than the termination of glaciations. Here an idea is proposed for the slower transition by identifying and describing two negative sea ice feedbacks dominant during the glaciation process that could serve as a control on the intermediate stage and decrease the pace of the process.
Paleoclimate data show that the Earth's climate of the last 2.6 Myr is dominated by cold glaciations; recently (after the Mid-Pleistocene Transition) the durations of these ice ages – with extensive glaciers – have been ∼100 kyr, with warm interglacials with little global ice cover lasting 10–30 kyr (Imbrie et al., 1992). The termination of a glacial period occurs rapidly while the changeover to a glacial period takes tens of thousands of years to be completed, resulting in an interesting asymmetrical shape for which there is yet no consensus on the mechanism(s) (Tziperman and Gildor, 2003). From benthic δ18O (‰) records from the Ocean Drilling Program (ODP) Site 983 in Raymo et al. (2004), the duration of the last termination was ∼10 kyr while the glaciation process including the inception and intermediate stage had a duration of ∼77 kyr. There is a plethora of previous research which has identified several feedback mechanisms to explain glacial–interglacial asymmetry, for example, varying ice sheet volumes (Le Treut and Ghil, 1983) or the sea ice switch mechanism (Gildor and Tziperman, 2000). While it is mostly agreed that astronomical forcings trigger glacial–interglacial transitions, a similar shape is not observed in insolation changes, suggesting a nonlinear response by the climate system (Lisiecki and Raymo, 2007). Gildor and Tziperman (2000) proposed a sea ice switch mechanism which says the sea ice acts as a control on the atmospheric moisture fluxes and precipitation through its albedo and insulating effects switching it between two modes, growing land ice and retreating land ice. A similar mechanism is presented here but differs in that it considers the effect of sea ice insulation on the temperature and stability of the deep ocean instead of land ice sheets. Sea ice cover is considered as a control on deep-ocean temperature in the Arctic Ocean, which in turn can control the extent of sea ice cover by vertical turbulence and lead to the development of an intermediary stage as illustrated in the schematic, Fig. 1.
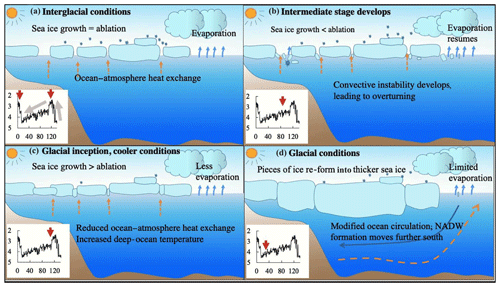
Figure 1Illustration showing how the proposed two negative sea ice feedbacks operate to slow down the glaciation process with an inset showing benthic δ18O (‰) records from ODP Site 983 (Raymo et al., 2004) spanning the last 140 kyr. The red arrows in the insets show the glacial transition stage described in the given panel. The grey arrows compare termination and glaciation transitions. (a) Interglacial conditions exist; there is strong exchange of energy (dashed orange arrows) and evaporation of moisture (blue arrows) from the ocean. (b) Initiation of glacial inception; sea ice extends, insulating the ocean while deep-ocean energy accumulates, increasing deep-ocean temperatures and buoyancy eventually creating an unstable water column. (c) At some critical point, when the water column becomes sufficiently unstable there is overturning and ablation of the young sea ice, albedo decreases and temperatures increases, creating the observed intermediary stage. (d) Sea ice has reformed due to continued cooler than interglacial conditions. This sea ice may be thicker and sturdier, better able to resist ocean turnover, leading to changes in the ocean circulation patterns instead of sea ice ablation.
Here it is proposed that the sea ice–precipitation feedback is one of the first of many feedbacks to become dominant during glaciations. Figure 1a shows possible conditions of the Arctic Ocean during an interglacial; there is little sea ice formation but strong energy and mass ocean–atmosphere exchange. After the glaciation process is initiated, temperatures cool in the Northern Hemisphere (NH), indicated by a drop in temperatures as observed in the inset of Fig. 1b. We think this allows sea ice to extend rapidly, increasing albedo and further decreasing temperatures, and helps to explain the initial rapid temperature drop observed for this glacial-inception period which lasts ∼10 kyr. It has been suggested that Arctic sea ice controls climate by regulating albedo and air–sea exchange of both energy and gases (Weyl, 1968). Both proxy and modeling data indicate significant ice cover over the Arctic Ocean during the Last Glacial Maximum (Colleoni et al., 2009; Jakobsson et al., 2016). The increased sea ice coverage and decreased air–sea exchange may have caused cold, dry atmospheric conditions to develop, reducing precipitation as depicted in Fig. 1b. With less precipitation in the Arctic region, there is less ice accumulation, and sea ice growth is hindered. Hydrogen isotope ratios (d-excess values) confirm that sea ice controls regional Arctic precipitation by contributing to drier conditions on Greenland as sea ice extent increases (Kopec et al., 2016). The extensive sea ice now formed becomes starved as precipitation and ice accumulation becomes limited; the newly formed sea ice thins and is more vulnerable to ablation. Enhanced ocean movements can result in rapid ablation as shown in Fig. 1c (the red arrow on the inset shows the stage in the transition). The loss of the sea ice reduces albedo and causes increased temperatures resulting in an intermediate stage with higher temperature or mild glacial conditions as described by Paillard (1998). Based on temperature reconstruction, the intermediate stage has a duration of ∼42 kyr, where there is increased evaporation, due to greater exposed ocean surfaces relative to the initial glacial-inception period, and increased precipitation. As overall NH temperatures remain cooler than during interglacials, with low insolation values prevailing, the sea ice eventually reforms into sturdier sea ice cover that is more resistant to ocean turnover (Fig. 1d).
The second dominant feedback proposed is sea ice–insulation feedback as portrayed in Fig. 1. Results from the atmospheric HIRHAM regional climate model showed sea ice acts to regulate air–sea heat flux by allowing stronger heat flux when sea ice thickness is reduced (Curry et al., 1995). With the formation of sea ice in the Arctic Ocean, as explained above and shown in Fig. 1b, and reduced air–sea energy exchange, there is reduced heat loss and geothermal energy buildup in the deep ocean, increasing seawater buoyancy gradually, and at some critical point there is rapid vertical ocean turbulence and sea ice ablation, illustrated in Fig. 1c. In a study of Dansgaard–Oeschger events, the ECBilt-CLIO model demonstrates the effect of sea ice on deep-ocean temperature where a 13–15×106 km2 sea ice extent led to the deep-ocean temperature increase of 2–4 ∘C at 1.5–3.5 km depth and a mean ocean temperature change of 2–5 ∘C (Rial and Saha, 2011). This induces instability with cold dense water at the surface and warmer water in the deep ocean resulting in turbulent vertical mixing at some point and sea ice ablation. The first proposed feedback sea ice–precipitation likely enhances this sea ice disintegration, where decreased precipitation results in thinning sea ice and increased susceptibility to perturbations such as turbulent mixing. Sea ice disintegration decreases albedo, resulting in a return to higher temperatures and the development of the intermediary stage of almost interglacial conditions depicted in Fig. 1c. By aiding the return to higher temperatures, the sea ice–precipitation feedback works as a negative feedback. The intermediary stage of reduced albedo and warmer conditions may have a wider influence by affecting other climate variables such as land-based ice sheets which can in turn impact the behavior of other dominant players in this climate transition. This stage remains dominant temporarily with sea ice cover formation increasing and ablation processes following until gradually sturdier sea ice forms as, overall, temperatures cooler than interglacial temperatures prevail as shown in Fig. 1d. The more durable sea ice has greater resilience to ocean turnover, and, instead of breaking up with ocean turbulence, there are changes in local North Atlantic convection sites. Shifting the position of the North Atlantic Deep Water (NADW) formation is demonstrated by previous studies, for example, Rahmstorf (2006).
Paillard (1998) showed the existence of an intermediate stage during glacial inception in which an ice volume threshold must be crossed before the transition to full glacial conditions can occur. Building on this concept, this paper proposes two negative feedbacks, sea ice–precipitation and sea ice–insulation, that explain the formation of an intermediary stage during glaciations, slowing the process. The dominance of these two feedbacks has implications for future replication of the climate dynamics of entrances into and exits from interglacials and for similar critical transitions of dynamical systems by emphasizing the role of negative feedbacks in regulating the speed of climate transitions.